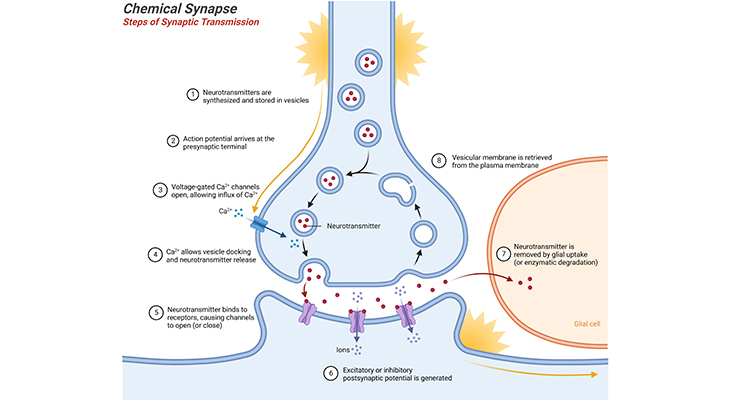
Figure 1. Chemical Synapse – Steps of Synaptic Transmission: Neurotransmitter release at the synapse, into the synaptic cleft. The synapse consists of various specializations where the presynaptic and postsynaptic neuronal membranes are close together. Neurotransmitters are synthesized and stored in vesicles. When the action potential arrives at the presynaptic axon terminals, it causes voltage-gated Ca2+ channels to open, allowing influx of Ca2+; triggering vesicles to bind to the presynaptic membrane. Neurotransmitter is released into the synaptic cleft by exocytosis and diffuses across the cleft. Binding of neurotransmitter to receptor molecules of postsynaptic neuronal membrane completes the process of transmission, i.e., either excitatory or inhibitory postsynaptic potential is generated depending on the type of neurotransmitter. Finally, the neurotransmitter is removed by glial uptake or enzymatic degradation, and the vesicular membrane is retrieved and/or recycled from the plasma membrane.
Introduction
Understanding the neurochemistry of autism is critically important for the development of effective physical treatments. This has been recognized for so many years, and the quest for the search for neurochemical abnormalities in autism, such as might be treatable by medication or dietary changes, began shortly after autism was identified as a distinct developmental disorder.
The primary goal of this blog and all the subsequent ones are to provide a succinct overview of what is known concerning the neurobiology, or brain bases, of autism, and at the same time indicate the likely growth areas of research. Nevertheless, in order to understand the neurobiological bases of autism spectrum disorders (ASD), it is necessary to have some minimal understanding of the neurotypical brain, i.e., some basic facts concerning the structure, function, and development of the neurotypical brain, before we delve into the changes that are concerning the structure, function, and development of the autistic brain. In this context, here we have presented an exclusive overview of neurotypical brain chemistry.
Synaptic Transmission – Chemical and Electrical
The human nervous system is composed of a larger number of separate neurons, and electrical signals cannot jump between cells like a static electricity generator. The terminal region of the neuronal axon (Greek axon = axis) is formed into a structure, the synapse, which is specialized for sending a chemical signal from one cell to the next. Neurons communicate with other neurons, with muscles, or with glands at synapses, and the process of transfer of a signal or impulse from the axon terminal of one neuron to the next neuron is called synaptic transmission. In fact, there are two major kinds of synapses – chemical and electrical – each using very distinct mechanisms for synaptic transmission [1, 11].
I. Chemical Synapse: Chemical Transmission
The majority of neurons send a signal to the cell across the synapse by releasing chemical neurotransmitters into the synaptic cleft, which is the gap between neurons at the synapse. The general mechanism that governs synaptic chemical transmission is as follows [1] (Figure 1; Box – 1):
- The neurotransmitters are synthesized and stored in small vesicles.
- The arrival of the action potential {an action potential is a rapid sequence of changes in the voltage across a membrane; i.e., a temporary shift, from negative to positive, in the neuronal membrane potential caused by ions suddenly flowing in and out of the neurons} at the axon terminal leads to depolarization of the terminal membrane, causing voltage-gated Ca2+ channels to open.
- The opening of these calcium channels triggers small vesicles containing neurotransmitter to fuse with the membrane at the synapse and release the transmitter into the synaptic cleft.
- Different types of neurons produce and release different neurotransmitters; some of them may release more than one type at a time, in what is sometimes called co-transmission.
- The transmitter diffuses across the cleft and, on reaching the postsynaptic membrane of the partnering neuron, binds with specific receptors that are embedded in it.
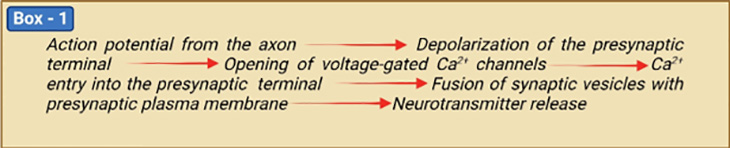
1. Two Distinct Types of Neurotransmitter Receptors
There are two types of ‘postsynaptic receptors’ [2 – 4] (Figure 2). Specific neurotransmitters bind to each type of postsynaptic receptor.
- Ligand-gated ion channels where neurotransmitter binding directly gates (opens) the ion channel.
- G protein-coupled receptors (GPCRs) where biochemical signals indirectly cause the gating of ion channels.
In ligand-gated ion channels, binding induces a conformation change in the receptor molecule. The change in shape opens an ion channel, resulting in an influx of ions leading to either depolarization (excitation) or hyperpolarization (inhibition) of the postsynaptic cell.
The metabotropic receptors require G proteins and second messengers to indirectly modulate ionic activity in neurons. Excitatory and inhibitory neurons are also capable of modulating functions through the GPCRs. G proteins are those that bind the guanine nucleotide GDP and GTP (guanosine di- and triphosphate) and act as molecular switches in cells. There are over 1,000 different GPCRs, indicating the complexity of the system. The specific GPCRs that are present depend on the neuron and where the neuron is located. Each type of GPCR is activated by a specific signaling molecule that could be a neurotransmitter, a neuropeptide (a small protein-like molecule secreted by neurons or glial cells), or a neurosteroid, among other possible signals.
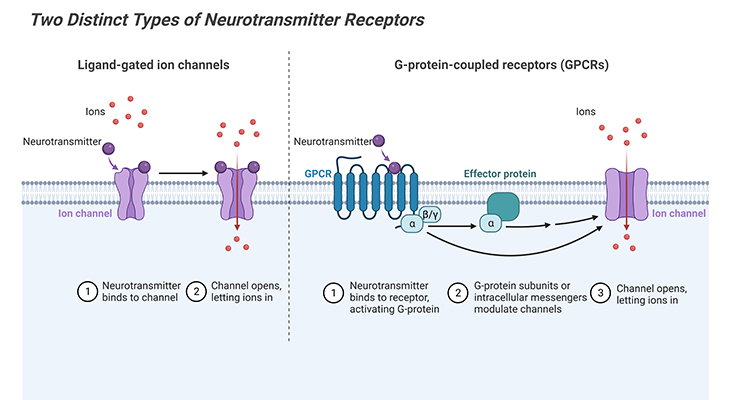
Figure 2. Two Distinct Types of Postsynaptic Neurotransmitter Receptors. Neurotransmitter receptors are either ionotropic or metabotropic. Ionotropic receptors are ion channels gated by neurotransmitter binding and act rapidly to produce synaptic potentials with a few milli seconds of presynaptic action potential arrival. For example, ionotropic acetylcholine and glutamate receptors are nonselective cation channels; upon neurotransmitter binding, these receptors produce depolarization in the form of excitatory postsynaptic potentials. All metabotropic receptors are G-protein-coupled receptors. Neurotransmitter binding activates trimeric G proteins associated with the receptors. Gα-GTP and Gβγ each can activate different effectors, depending on G protein variants and cellular contexts. Gβγ can act on K+ and Ca2+ channels directly, whereas Gα usually acts via second messengers such as cAMP and Ca2+ to activate protein kinases that phosphorylate ion channels to change the membrane potential and excitability of the postsynaptic neurons. Metabotropic receptor activation causes membrane potential changes within tens of milliseconds to seconds.
When a signaling molecule specifically binds to its GPCR, the conformational change activates a G protein withing the cell, which in turn activates or regulates a specific target protein, typically an enzyme, which produces a diffusible molecule of some sort called a second messenger. The second messenger, in turn, triggers a biochemical cascade of reactions.
While directly gated channels mediate fast signaling, measured in milliseconds, GPCR-mediated signaling is slower, occurring over hundreds of milliseconds or even seconds, and thus producing longer-lasting modulatory changes to the functional state. For example, the neurotransmitter epinephrine (adrenaline) binds to a particular GPCR. Once bound, a G protein is activated that seeks out the protein adenylate cyclase and activates it. The activated adenylate cyclase turns ATP into cAMP (cyclic adenosine monophosphate), which acts as a second messenger of information instructing the postsynaptic neuron.
2. Inactivation of Neurotransmitters after Release
Following the release of neurotransmitter into the synaptic cleft and its binding with the postsynaptic membrane receptors, the remining must be removed to prevent further excitatory or inhibitory signal transduction [1, 7]. This removal is crucial and can be accomplished by:
- Active reuptake of the substance back into the presynaptic terminal (e.g., biogenic amines – dopamine, norepinephrine, epinephrine, serotonin, and histamine), or
- By enzymatic breakdown of the neurotransmitter in the synaptic cleft (e.g., acetylcholine), or
- Merely diffusion of the neurotransmitter away from the region of the synapse or site of action (e.g., in the case of hormones that act on target cells distant from the synaptic terminals).
Presynaptic neurons have the so-called ‘autoreceptors’ that monitor the level of neurotransmitter in the synaptic cleft. These autoreceptors are located on the presynaptic terminals and bind with the released transmitter, enabling the presynaptic neurons to regulate the synthesis and release of the transmitter, via either a negative or positive feedback processes.
3. Neurochemicals
Neurochemicals in the brain fall into various but sometimes overlapping categories according to their major function or functions [5 – 7]. Three important groupings of brain chemicals are, viz., (a) neurotransmitters, (b) neuromodulators, (c) neurotrophins.
(a) Neurotransmitters: are chemical substances released by one neuron to either stimulate or inhibit activity in other neurons. Neurotransmitters primarily act as ‘messengers’ carrying ‘information’ from one neuron to other neurons equipped with specialized neuroreceptors selectively responsive to the transmitter substance.
While one may have heard of a few of the classic neurotransmitters, more than 100 have been identified within the human body. Some of them operate only within the peripheral nervous system (PNS) with highly specialized functions pertaining to, for instance, digestion or circulation. However, other neurotransmitters operate in both the peripheral and central nervous system (CNS) with a wide range of functions [9 – 10] (Figure 3;
Table 1).
What are defining characteristics of a neurotransmitter? or specifically, what makes a molecule a neurotransmitter?
- Neurotransmitter is synthesized and localized with the presynaptic neuron; and stored in the presynaptic terminal before release.
- Neurotransmitter is released by the presynaptic neuron when action potentials depolarize the terminal (mediated primarily by Ca2+).
- The postsynaptic neuron contains receptors specific for neurotransmitter.
- Besides, when artificially applied to a postsynaptic cell, it elicits the same kind of response that stimulating the presynaptic neuron would.
(b) Neuromodulators: are substances that either stimulate or depress the chemical output from neurons (comparable to volume control on a television set). All of the neurotransmitters listed above in Table 1 and Figure 3 can also act as neuromodulators. For example, serotonin, dopamine, acetylcholine and norepinephrine (aka UK: noradrenalin) modulate neural activity within their own specialized brain circuits, in general, referred to as the dopaminergic, cholinergic, adrenergic, and serotoninergic systems, respectively (Figure 4 – 5). Glutamate and γ-amino butyric acid (GABA), on the contrary, are active thought the brain, with significant roles in the excitation and inhibition of neural activity. Apart from this, certain other neuromodulators are classified primarily as hormones rather than as neurotransmitters, which include, for example, cortisol, testosterone, oxytocin, and vasopressin.
(c) Neurotrophins: are involved in brain growth and maintenance. The most important one is brain derived neurotropic factor (BDNF), which is active in the brain from conception onwards. As well as both serotonin and GABA also act as neurotrophins prenatally, as does reelin.
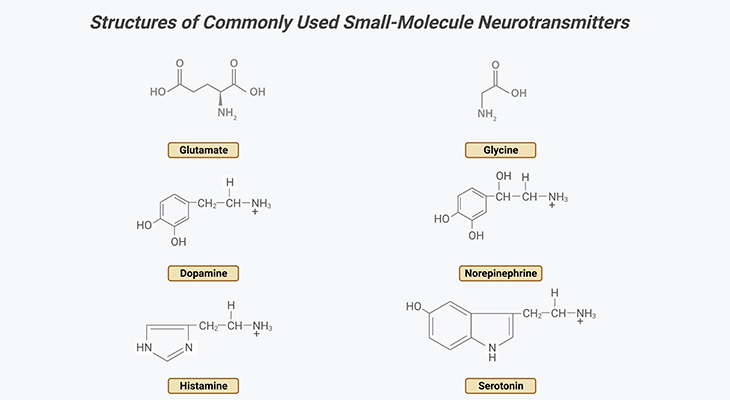
Figure 3. Structures of Commonly Used Small-Molecule Neurotransmitters. Glutamine and glycine are natural amino acids. GABA (γ-amino butyric acid) is produced from glutamate. Dopamine is derived from the amino acid tyrosine. Norepinephrine is produced from dopamine and is a precursor molecule for the hormone epinephrine. Histamine is derived from the amino acid histidine. Serotonin, also called 5-HT for 5-hydroxytryptamine.
4. Functions of Some Major Neurochemicals in the Human Brain
Neurotransmitter | Major Uses in the Human Nervous System |
Glutamate | Glutamate Most CNS excitatory neurons; most sensory neurons |
GABA | Most CNS inhibitory neurons |
Dopamine | CNS modulatory neurons |
Acetylcholine | Motor neurons that excite muscle; CNS excitatory and modulatory neurons; ANS neurons |
Norepinephrine | CNS modulatory neurons; ANS neurons |
Serotonin (5-HT) | CNS modulatory neurons; neurons in the gastrointestinal tract |
Histamine | CNS modulatory neurons |
Glycine | Some CNS inhibitory neurons (mostly in the brain stem and spinal cord) |
ATP; Adenosine | CNS neurons; Some sensory neurons |
Neuropeptides | Usually co-released from excitatory, inhibitory, or modulatory neurons; neurosecretory cells |
CNS, central nervous system; ANS, autonomic nervous system (acetylcholine and norepinephrine are used in different types of ANS neurons. |
Table 1. Commonly used neurotransmitters.
i. Glutamate: the principle players in the balancing act between excitation and inhibition are glutamate and GABA. Glutamate is released by the pyramidal cells of cortex, the most frequent cortical neurons [8]. A few different types of receptors bind glutamate, some of these are found in modifiable synapses (i.e., ones that can change in strength) involved in learning and memory. Excessive glutamate (excitation) can be toxic and lead neuronal death; and has been implicated in neurodegenerative disorders such as Parkinsons’s and Alzheimer’s disease, as well as in epilepsy and stroke.
ii. γ-amino butyric acid (GABA): is the second most common neurotransmitter and is synthesized from glutamate. It is found in most of the fast inhibitory synapses throughout the brain; and exerts inhibitory control on neural activity throughout the brain, acting in synergy with glutamate, which exerts excitatory control. A balance between the activities of GABA and glutamate is essential to achieve adaptive control of muscle tone and movement, as well as several cortical functions, including vision and arousal. Decreased expression levels of GABA (decreased inhibition) can result in seizures, as well as increases in emotional reactivity, sweating, heart rate, blood pressure, food and water intake, insulin secretion, gastric acid, and colonic motility. Above all, too much GABA can lead to coma.
iii. Serotonin: in the brain is released predominantly by the neurons of the raphe nuclei, located in the brainstem. Serotonin has both excitatory and inhibitory functions. Serotonin plays a role in the development of brain cells and their connections; as well as in the organization of brain growth prenatally [9 – 10]. It is for this reason that it is considered inadvisable for pregnant women to be prescribed medications designed to induce serotoninergic activity. Drugs such as the selective serotonin reuptake inhibitors (SSRIs), used to treat clinical depression, act on the raphe nuclei and their targets in the brain. Serotonin plays a major role in digestion, as well contributing to the regulation of blood pressure, temperature, appetite, behavior, muscle contraction, pain thresholds, mood, sleep, as well endocrine systems. Furthermore, serotonin also has effects on cognitive functions, including memory and learning (Figure 4).
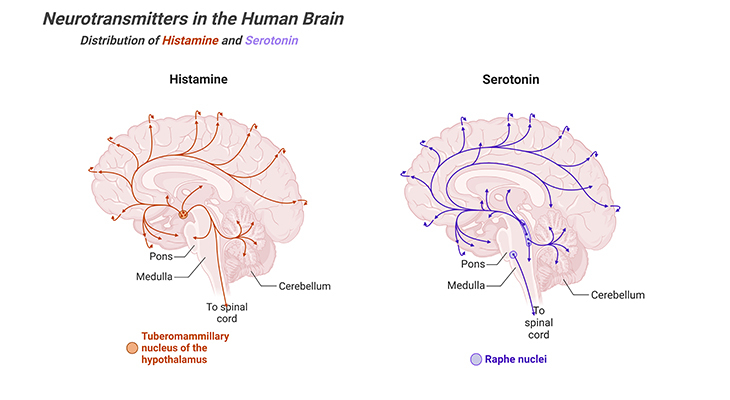
Figure 4. Distribution of Histamine and Serotonin Neurotransmitters in the Human Brain. Major projection pathways of the biogenic amine neurotransmitter systems in the human brain. Shown are the projections of the histamine and serotonin systems using the neurotransmitters. The views are midsagittal sections through the human brain, depicting the medial surface of the right hemisphere; the front pole is at left. In each image, the primary source of the biogenic amine is bold type and colored accordingly.
iv. Dopamine: the cardinal sites of dopamine production are adrenal glands and a few small regions of the brain. Brain areas with significant dopaminergic innervations include the striatum, substantia nigra, and hypothalamus (Figure 5). Thus far, five different types of dopamine receptors have been identified, all of them are GPCRs. Dopaminergic pathways are involved in particular functions, for instance, cognitive and motor control, motivation, arousal, reinforcement, and reward or ‘feel-good factors’ (most recreational drugs act via dopaminergic reward systems), among others. Deficits in dopamine systems are associated with schizophrenia, attention deficit hyperactivity disorders (ADHD), addiction, and most importantly Parkinson’s disease.
v. Norepinephrine: also known as noradrenaline, is a sympathetic nervous system’s go-to neurotransmitter. It is produced and utilized by neurons with cell bodies in the locus coeruleus (LC), an area of the brain involved with physiological responses to stress and located in one of the brainstem’s structures, the pons. In the CNS, it has a role in the maintenance of sustained attention and in this way contributes to learning (Figure 5).
Outside the brain, norepinephrine is released by the adrenal glands; mediates ‘flight-or-fight response.’ It increases the arousal, alertness, and vigilance; focuses attention; and enhances memory function. Norepinephrin is involved in autonomic nervous systems (ANS) functions, including the neurochemical correlates of fear and anxiety, such as sweating, increased heart rate, blood pressure, and blood flow to skeletal muscles, and decreased blood flow to the gastrointestinal systems. It also increases the availability of stored glucose for energy.
vi. Acetylcholine (ACh): is also mainly involved in ANS functions, initiating involuntary muscle activity. (e.g., digestion) in particular, and stimulating the excretion of certain hormones. In the CNS, Ach acts as a neurotransmitter and a neuromodulatory and supports cognitive functions. It binds to two main types of receptors, nicotinic and muscarinic, that have different properties and functions. It contributes to wakefulness and arousal, sustain attention, enhance learning and memory, mood (including aggression), sexuality, thirst, and increase in REM (rapid eye movement) sleep. Ach is present at the neuromuscular junctions, where it has excitatory activity and activates muscles.
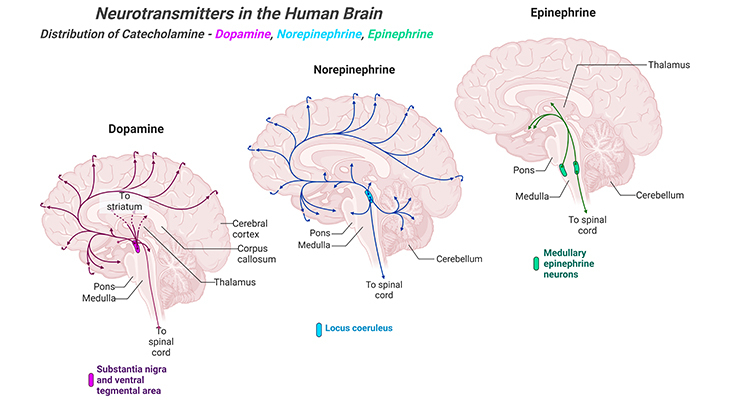
Figure 5. Distribution of Catecholamine Neurotransmitters in the Human Brain. Major projection pathways of the biogenic amine neurotransmitter systems in the human brain. Shown are the projections of the dopamine, norepinephrine, and epinephrine systems using the neurotransmitters. The views are midsagittal sections through the human brain, depicting the medial surface of the right hemisphere; the front pole is at left. In each image, the primary source of the biogenic amine is bold type and colored accordingly.
vii. Brain-derived neurotrophic factor (BDNF): is critically involved in the production, differentiation, and positioning of neurons in the brain (and elsewhere in the body) prenatally. Consequently, it supports the survival of existing neurons, and stimulates the growth of new neurons and synaptic connections in the brain. Thus, having a crucial role in neuronal plasticity and memory.
viii. Reelin: in combination with BDNF and serotonin, contributes to prenatal brain development.
ix. Oxytocin: modulates activity within brain circuits mediating pain perception, emotion, appetite, sexual behavior and social bonding. It also has a major role in mediating bodily functions associated with pregnancy, childbirth, and breast feeding.
x. Testosterone: is primarily associated with male sexuality, however, may also be associated with certain cognitive abilities, including spatial sense and some facets of memory/learning.
xi. Vasopressin: has significant roles in certain autonomic functions, including water retention. Recently, it has been suggested that it may have a certain role in mediating social engagement and reward, but this is not proven.
xii. Cortisol: produces bodily changes in response to stress (i.e., ‘fight or flight readiness’); variations in cortisol levels are associated with sleep-wake cycle. It also has an anti-inflammatory role within the immune system.
II. Electrical Synapse: Electrical Transmission
Unlike chemical synapses, few neurons communicate through electrical synapses. In electrical synapses, there is no synaptic cleft that separates the pre- and postsynaptic neurons. Rather, the neuronal membranes touch at specialized cell junctions, the so-called gap junctions; and the cytoplasms of two partnering neurons are essentially continuous. As a result, these gap junction channels create pores that are composed of multiple transmembrane proteins such as connexons, connecting the cyptoplams of the two neurons. Consequently, the two neurons are isopotential, implying that electrical changes in one are reflected instantaneously in the other [Figure 6] [11].
Electrical synapses are useful when information must be relayed rapidly, for instance in the escape reflex of some invertebrates. Groups of neurons with these synapses can activate muscles quickly to get the animal out of harm’s way. A more general purpose of electrical synapses is to synchronize electrical activity among populations of neurons; or when groups of neurons should operate synchronously, as with some hypothalamic neurosecretory neurons. Thus, facilitating a burst of hormone secretion into the circulation.
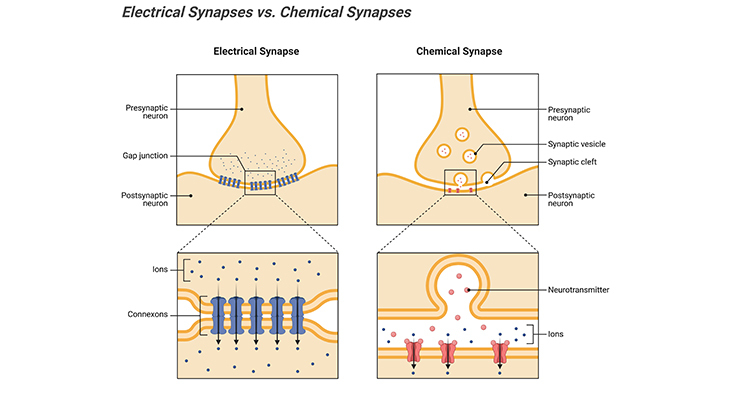
Figure 6. Electrical Synapses versus Chemical Synapses Between Two Neurons. Electrical synapses are formed by gap junctions, places where multiple transmembrane proteins such as connexons in the pre- and postsynaptic neurons connect to create pathways that connect the cytoplasm of the two neurons. Under most circumstances, the communication is bidirectional, however, so-called rectifying synapses limit current flow in one direction. In chemical synapse, the pre- and postsynaptic neuronal membranes are separated by a synaptic cleft, a fluid filled space. The chemical event is involved in the transmission of impulse via release, diffusion, receptor binding of neurotransmitter molecules and unidirectional communication between neurons.
The electrical synapses also have certain limitations. For example, unlike chemical synapses, electrical synapses are much less plastic, and they cannot amplify a signal; while an action potential that triggers a chemical synapse could potentially cause a large burst of release of neurotransmitter, thus amplifying the signal.
Summary and Conclusions
In this blog we have given a brief account of the neurochemistry of the neurotypical brain and described the expression of some commonly encountered neurotransmitters and their functions; as well as their modes of transmission via chemical and electrical synapses.
Synapses are the locations where one neuron can transfer information to another neuron or a specialized non-neuronal cell. Synapses are found not only on dendrites and at axon terminals but can also be found on the neuronal cell body.
Chemical transmission results in the release of neurotransmitters from the presynaptic neuron and following the binding of those neurotransmitters on the postsynaptic neuronal receptors, which in turn causes either excitatory or inhibitory postsynaptic potentials (EPSPs, excitatory postsynaptic potentials or IPSPs, inhibitory postsynaptic potentials), depending on the properties of the postsynaptic receptor.
Neurotransmitters must be removed from the receptor after binding. This removal can be accomplished by one of the following three mechanisms, active reuptake back into the presynaptic terminal; enzymatic breakdown of the transmitter in the synaptic cleft; or diffusion of the neurotransmitter away from the region of the synapse.
Electrical synapses are different from chemical synapses because they operate by passing current directly from one neuron to another neuron, i.e., from presynaptic to postsynaptic neuron, via specialized channels in gap junctions that connect the cytoplasm of one cell directly to the other.
For information on autism monitoring, screening and testing please read our blog.
References
- Chanaday NL, Cousin MA, Milosevic I, Watanabe S, Morgan JR. The Synaptic Vesicle Cycle Revisited: New Insights into the Modes and Mechanisms. J Neurosci. 2019 Oct 16;39(42):8209-8216. doi: 10.1523/JNEUROSCI.1158-19.2019. PMID: 31619489; PMCID: PMC6794917.
https://pubmed.ncbi.nlm.nih.gov/31619489/ - Humphrey PP. The characterization and classification of neurotransmitter receptors. Ann N Y Acad Sci. 1997 May 30;812:1-13. doi: 10.1111/j.1749-6632.1997.tb48141.x. PMID: 9186716.
https://pubmed.ncbi.nlm.nih.gov/9186716/ - Hille B. Ion Channels of Excitable Membranes. 3rd ed. Sinauer Associates Is an Imprint of Oxford University Press; Sunderland, MA, USA: 2001.
https://global.oup.com/academic/product/ion-channels-of-excitable-membranes-9780878933211?cc=us&lang=en&# - Smith RS, Walsh CA. Ion Channel Functions in Early Brain Development. Trends Neurosci. 2020 Feb;43(2):103-114. doi: 10.1016/j.tins.2019.12.004. Epub 2020 Jan 17. PMID: 31959360; PMCID: PMC7092371.
https://pubmed.ncbi.nlm.nih.gov/31959360/ - Sheffler ZM, Reddy V, Pillarisetty LS. Physiology, Neurotransmitters. 2023 May 1. In: StatPearls [Internet]. Treasure Island (FL): StatPearls Publishing; 2023 Jan–. PMID: 30969716.
https://pubmed.ncbi.nlm.nih.gov/30969716/ - Hyman SE. Neurotransmitters. Curr Biol. 2005 Mar 8;15(5):R154-8. doi: 10.1016/j.cub.2005.02.037. PMID: 15753022.
https://pubmed.ncbi.nlm.nih.gov/15753022/ - Kavalali ET. The mechanisms and functions of spontaneous neurotransmitter release. Nat Rev Neurosci. 2015 Jan;16(1):5-16. doi: 10.1038/nrn3875. PMID: 25524119.
https://pubmed.ncbi.nlm.nih.gov/25524119/ - Zhou Y, Danbolt NC. Glutamate as a neurotransmitter in the healthy brain. J Neural Transm (Vienna). 2014 Aug;121(8):799-817. doi: 10.1007/s00702-014-1180-8. Epub 2014 Mar 1. PMID: 24578174; PMCID: PMC4133642.
https://pubmed.ncbi.nlm.nih.gov/24578174/ - Herlenius E, Lagercrantz H. Neurotransmitters and neuromodulators during early human development. Early Hum Dev. 2001 Oct;65(1):21-37. doi: 10.1016/s0378-3782(01)00189-x. PMID: 11520626.
https://pubmed.ncbi.nlm.nih.gov/?term=Herlenius+E&cauthor_id=11520626 - Teleanu RI, Niculescu AG, Roza E, Vladâcenco O, Grumezescu AM, Teleanu DM. Neurotransmitters-Key Factors in Neurological and Neurodegenerative Disorders of the Central Nervous System. Int J Mol Sci. 2022 May 25;23(11):5954. doi: 10.3390/ijms23115954. PMID: 35682631; PMCID: PMC9180936.
https://pubmed.ncbi.nlm.nih.gov/35682631/ - Connors BW, Long MA. Electrical synapses in the mammalian brain. Annu Rev Neurosci. 2004;27:393-418. doi: 10.1146/annurev.neuro.26.041002.131128. PMID: 15217338.
https://pubmed.ncbi.nlm.nih.gov/15217338/