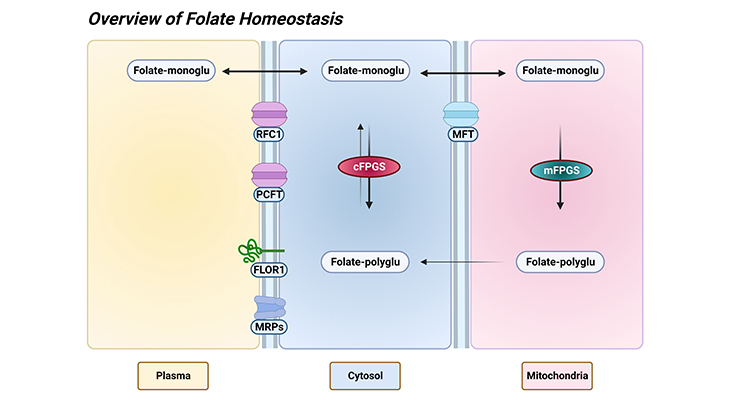
Figure 1. Overview of Folate Homeostasis. ‘Homeostasis’ refers to any process that living things use to actively maintain fairly stable conditions necessary for survival. In this connection, tissue folate homeostasis/accumulation requires folate metabolism to polyglutamate derivatives in the cytosol as well as mitochondria of human cells. The mitochondrial folate transporter (MFT) competes with the cytosolic folylpolyglutamate synthetase (cFPGS) for the entering folate monoglutamate. After transport into tissues: (a) monoglutamate derivatives are interconverted to other monoglutamates through the enzymes of one-carbon metabolism, or (b) some folates are metabolized to polyglutamate derivatives and retained, or (c) any not metabolized to longer-chain polyglutamate species are lost from the cells. A substantial proportion of cellular folate, up to 50% depending on the tissue or cell type, is associated with mitochondria. Besides, the mitochondrial folate pool is distinct from the cytosolic pool and exhibits a distinct one-carbon distribution. [Folate-monoglu – folate monoglutamate; Folate-polyglu – folate polyglutamate; FPGS – folylpolyglutamate synthetase (mitochondrial and cytosolic isoenzymes); FLOR1 – folate receptor alpha (folate binding proteins); MFT – mitochondrial folate transporter; MRPs – multidrug resistance proteins; PCFT – proton coupled folate transporter; RFC1- reduced folate carrier 1.]
Introduction
Homeostasis (Greek words for ‘same’ and ‘steady’), any self-regulating process by which biological systems tend to maintain stability. The stability attained is actually a dynamic equilibrium, in which continuous change occurs yet relatively uniform conditions prevail.
In this context, the ability of tissues to accumulate folate depends on several factors such as: (a) the level of and specificity of the folate transporter in the tissue, and (b) the level of folylpolyglutamate synthetase activity. Both of these processes will be influenced by: (a) folate intake and status, and (b) the levels of intracellular folate-binding proteins. Under certain conditions, transport would be limiting; and under other conditions, metabolism to retainable polyglutamates becomes limiting.
I. Folate One-Carbon Journey: From Food to Macromolecules
Here we provide a succinct overview of folate one-carbon journey from food to macromolecules. Tracing the pathway of folates ingested and the various folate transporter systems that mediate folate transport across various types of epithelia and into systemic tissues (Figure 1).
1. Hydrolysis of Folate Polyglutamates:
First, the major natural folate forms in the diet are polyglutamate congeners of 5-methyl tetrahydrofolate (THF), which are obviously not suitable substrates for the absorptive process. This implies, rather, folate polyglutamate derivatives must be hydrolyzed to their corresponding monoglutamate forms by the enzyme glutamate carboxypeptidase II (FOLH1, folate hydrolase 1) in human intestine, upon ingestion. This enzyme reaction has a low pH optimum similar to the absorptive process, consistent with the low pH at the surface of mucosal cells (enterocytes) in the proximal part of the jejunum [1] (cf. previous blog entitled as ‘Intestinal Absorption of Dietary Folates ‘)
2. Intestinal Absorption of Monoglutamates:
Secondly, once folate monoglutamate is generated by the hydrolytic process, it is transported across the apical brush-border membrane of the proximal jejunum mediated by proton-coupled folate transporter (PCFT; SLC46A1 – solute carrier family 46 member 1). PCFT transport is concentrative, driven by the transmembrane proton gradient generated by Na+/H+ exchangers, facilitating folate monoglutamate transport through the intestinal membranes into the enterocytes, i.e., folate influx. Consequently, the high folate levels in enterocytes should facilitate folate efflux across the basolateral membrane into the periserosal space, and from there, folates enter the vascular system.
The mechanism of export from enterocytes is not clear. Because neither reduced folate carrier (RFC1; SLC19A1 – solute carrier family 19 member 1) nor PCFT is expressed at the basolateral membrane. However, multidrug-resistant-associated proteins (MRPs) are expressed at this site, particularly MRP3 and/or MRP5 (ABCC3 and ABCC5 – ATP binding cassette subfamily C member 3 and 5) and might represent the route of export of folates [2] (Figure 2).
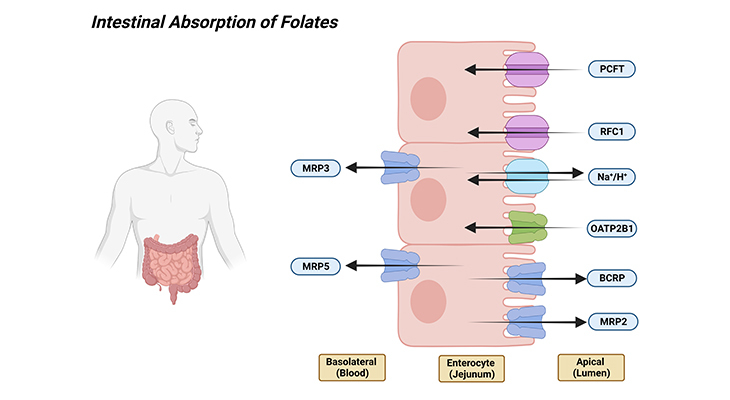
Figure 2. Intestinal Absorption of Folates. Shown are the various folate transporters that are expressed in the apical brush-border and basolateral membranes of the enterocytes. [PCFT – proton coupled folate transporter (solute carrier family 46 member 1); RFC1 – reduced folate carrier 1 (solute carrier family 19 member 1); Na+/H+ – sodium/hydrogen exchanger; OATP2B1 – solute carrier organic anion transporter family member 2B1; BCRP – ATP-binding cassette subfamily G member 2 [junior blood group]; MRP2, 3, and 5 – ATP binding cassette subfamily C members 2, 3, and 5.]
3. Enterohepatic Circulation of Folates:
Thirdly, after intestinal absorption, folates (mostly, monoglutamates) enter the hepatic portal system and are delivered to the liver, where it is either: (a) metabolized to polyglutamate derivatives and retained, or (b) it is released back into blood or bile. Folate transport in hepatocytes is energy-dependent and complex with saturable and non-saturable components.
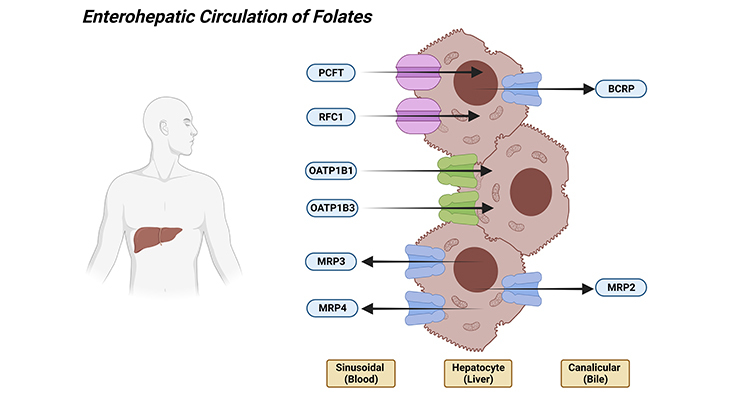
Figure 3. Enterohepatic Circulation of Folates. Shown are the various folate transporters that are expressed in the apical bile canalicular and basolateral membranes of the hepatocytes. [PCFT – proton coupled folate transporter (solute carrier family 46 member 1); RFC1 – reduced folate carrier 1 (solute carrier family 19 member 1); OATP1B1, and 1B3 – solute carrier organic anion transporter family member 1B1 and 1B3; BCRP – ATP-binding cassette subfamily G member 2 [junior blood group]; MRP2, 3, and 4 – ATP binding cassette subfamily C members 2, 3, and 4.]
PCFT is highly expressed in the liver, and it has been suggested that it localizes to the sinusoidal membrane. And this is, in consistent with a dominant low-pH folate transport activity encountered in hepatocytes and in sinusoidal membrane vesicles derived from hepatocytes. The concentrative folate transport is generated with a transvesicular pH gradient. Besides, even though RFC1 is localized at the hepatocyte membrane, its expression levels are low because there is minimal transport activity at pH 7.4 (Figure 3).
The net intestinal absorption of folates not only is determined at the intestinal level but also is governed by the flow of folates from the liver via the bile back into the intestine. This process is mediated mostly by the superfamily of ATP-binding cassette (ABC) transporters, for example, MRP2 (ABCC2 – ATP binding cassette subfamily C member 2) and BCRP (ABCG2 – ATP binding cassette subfamily G member 2 [junior blood group]), which are expressed in the apical bile canalicular membrane.
As well as by liver-specific members of the organic anion transporter family such as OATP1B1 (SLCO1B1 – solute carrier organic anion transporter family member 1B1) and OATP1B3 (SLCO1B3 – solute carrier organic anion transporter family member 1B3), which are expressed in the basolateral membrane of the hepatocyte, in apposition to the hepatic sinusoid that receives blood from the hepatic portal vein and the hepatic artery.
Briefly, folates that enter the liver have three potential destinations [2]:
- Folates can be converted into polyglutamate storage forms, or
- They can be secreted into the bile at the hepatic canalicular membrane mediated by MRP2/BRCP, whereby they return to the duodenum and jejunum and are subsequently reabsorbed, thus complete the cycle of enterohepatic circulation, or
- Folate monoglutamates, formed by hydrolysis of polyglutamate forms stored in hepatocytes, or delivered directly from the hepatic portal vein, can enter the hepatic vein, ultimately reaching the systemic circulation whereby they accumulate in and meet the one carbon requirements of, peripheral tissues.
Biliary excretion of folate has been estimated at up to 100 μg/day in humans. Much of this would be reabsorbed in the small intestine, but loss of folate through this route could be significant in ‘malabsorption syndrome.’
4. Transport into Systemic Tissues:
Next, the membrane transport of folates into systemic tissues by the arterial system, where the pH is 7.4, is accomplished by the RFC1 (it is a transporter of folate and is involved in the regulation of intracellular concentrations of folate). Even though PCFT is often coexpressed with RFC1, PCFT function would be minimal and possibly may not contribute to the delivery of folate systemically. Unless there are tissues where acid microenvironment is generated by Na+/H+ exchangers at transport interfaces. As a result, transport into peripheral tissues primarily uses RFC1 [2].
Folates that are present in systemic circulation also encounter epithelial barriers separating compartments that encase high folate-dependent tissues. Notable examples are:
- Neural tissue isolated by the vascular (i) blood-brain barrier (BBB), and (ii) blood-CSF barrier.
- Placenta linking mother and fetus (iii) blood-placental barrier.
5. Folate Excretion (Filtration and Reabsorption in the Kidney):
Finally, blood folates not bound to serum proteins are freely filtered at the glomerulus and are reabsorbed in the proximal rental tubule [3]. However, because of a highly efficient reabsorptive mechanism, little or none is lost in the urine at physiological levels of folate intake. The net effect is that most of the secreted folate is reabsorbed. The renal clearance of folate derivatives is inversely proportional to their affinities for the folate-binding protein in the kidney proximal tubules. Although urine does not contain some folate derivatives, the bulk of the excretion products in humans are folate cleavage products.
The initial step in reabsorption involves tight binding of folate to FOLR1 (folate receptor alpha, FR-α) that is highly expressed at the luminal apical brush-border membrane of proximal tubules. This is followed by internalization of receptors, release of folate into the cytoplasm and export at the basolateral membrane by RFC1, where the pH is optimal for carrier function. The role of PCFT in renal folate reabsorption is not clear. Several SLC21 (SLCO-superfamily of transporters; formerly known as SLC21 – organic anion transporting polypeptides, OATPs) solute carriers are expressed at the apical brush-border membrane of proximal renal tubule cells and probably contribute to renal folate absorption, particularly at high folate loads, where FOLR1-mediated absorption is saturated [2] (Figure 4).
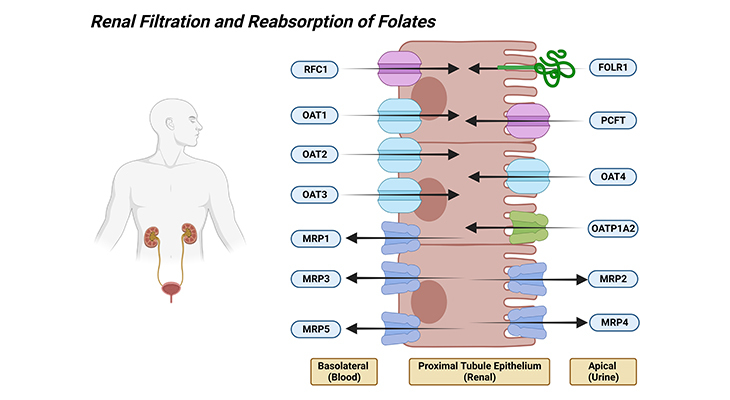
Figure 4. Renal Filtration and Reabsorption of Folates. Shown are the various folate transporters that are expressed in the luminal brush-border and basolateral membranes of the renal proximal tubular epithelial cells. [PCFT – proton coupled folate transporter (solute carrier family 46 member 1); RFC1 – reduced folate carrier 1 (solute carrier family 19 member 1); FOLR1 – folate receptor alpha; OATP1A2 – solute carrier organic anion transporter family member 1A2; MRP1, 2, 3, 4 and 5 – ATP binding cassette subfamily C members 1, 2, 3, 4, and 5; OAT1, 2, 3, and 4 – solute carrier family 22 members 6, 7, 8, 11, and 10.]
II. Tissue Accumulation
1. Endogenous Folates:
Most dietary folates are polyglutamate derivatives and are hydrolyzed to monoglutamate forms in the gut before absorption across the intestinal mucosa. Pteroylmonoglutamates, principally 5-methyl-tetrahydrofolate (5-methyl-THF), are the circulating forms of folates in plasma, furthermore, mammalian cells are not believed to transport polyglutamates of chain length three and above.
Endogenous folates in mammalian tissues, including human beings, are almost completely folylpolyglutamate derivatives, while pteroylmonoglutamates are the only forms in plasma and urine (Figure 1) [4]. Even though pentaglutamates are the predominant derivatives found in rat liver, longer chain derivatives are observed in most other mammalian tissues, including human cells.
This indicates that intracellular metabolism to polyglutamates is a prerequisite for the retention and concentration of transported folate. Though metabolism to polyglutamate derivatives might be considered a mechanism for intracellular folate storage, considering the fact that, folylpolyglutamates are the active coenzyme species, and metabolism to polyglutamates is essential for normal one-carbon metabolism. Consequently, there is no real store for excess folate.
Tissue folate accumulation that is summarized in Figure 1 (overview of folate homeostasis), requires folate metabolism to polyglutamate derivatives in the cytosol as well as mitochondria of human cells. Mitochondrial folate transporter (MFT) competes with the cytosolic folylpolyglutamate synthetase (cFPGS) for the entering folate monoglutamate [5]. After transport into tissues:
- Monoglutamate derivatives are interconverted to other monoglutamates through the enzymes of one-carbon metabolism.
- Some folates are metabolized to polyglutamate derivatives and retained.
- Any not metabolized to longer-chain polyglutamate species are lost from the cells.
Folate monoglutamates compete very poorly with cellular folylpolyglutamates for the enzymes of one-carbon metabolism. As a result, the extent of metabolism of monoglutamates will depend on the intracellular pools of folylpolyglutamates. Likewise, the ability to metabolize folates to retainable polyglutamate derivatives will be inhibited by competition with cellular folate pools.
2. Folate Distribution:
A substantial proportion of cellular folate, up to 50% depending on the tissue or cell type, is associated with mitochondria [6] (Figure 1). Besides, the mitochondrial folate pool is distinct from the cytosolic pool and exhibits a distinct one-carbon distribution.
- Essentially all the cellular 5-methyl-THF polyglutamate is in the cytosol.
- While most of the cellular 10-formyl-THF polyglutamate is in the mitochondria.
- Mitochondria contain folylpolyglutamates, which are of longer glutamate chain length than cytosolic folates.
Cells defects in mitochondrial folate metabolism contain a higher proportion of their mitochondrial and cytosolic folate pools as unsubstituted THF and are defective in purine synthesis and homocysteine remethylation.
The nucleus also contains a folate poll that is utilized for thymidylate synthesis. It is not clearly known how the nuclear folate pool is generally established, but it may be in equilibrium with the cytosolic folate pool.
3. Role of Folylpoly-γ-Glutamate Synthetase (FPGS):
FPGS gene encodes the folylpolyglutamate synthetase enzyme. This enzyme has a central role in establishing and maintaining both cytosolic and mitochondrial folylpolyglutamate concentrations, and therefore, is vital for folate homeostasis and the survival of proliferating cells. This enzyme catalyzes the ATP-dependent addition of glutamate moieties to folate and folate derivatives. Alternative splicing results in transcript variants encoding different isoforms, i.e., folylpolyglutamate synthetase is encoded by a single human gene. And cytosolic and mitochondrial isozymes are generated by alternate transcription start sites for the gene, and by alternate translational start sites for its mRNA [7] (Figure 1; Figure 5).
FPGS catalyzes conversion of folates to polyglutamate derivatives allowing concentration of folate compounds in the cell and the intracellular retention of these co-factors, which are important substrates for most of the folate-dependent enzymes that are involved in one-carbon transfer reactions involved in purine, pyrimidine, and amino acid synthesis. Unsubstituted reduced folates are the preferred substrates. Besides, FPGS metabolizes methotrexate (MTX) to polyglutamates.
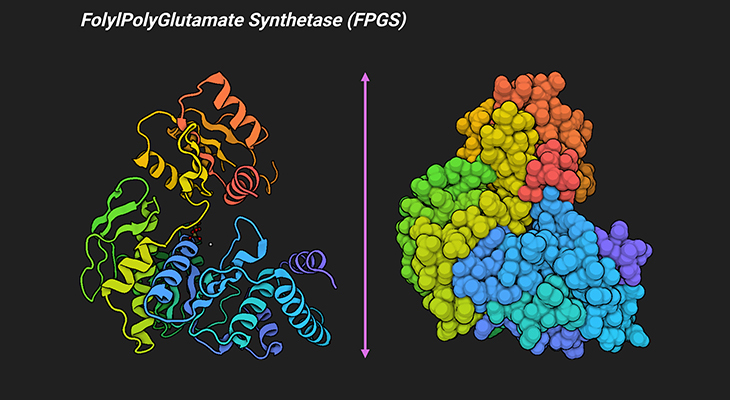
Figure 5. Three-Dimensional Structure of Folylpolyglutamate Synthetase (FPGS) from Lactobacillus casei. Ribbon diagram of a single Lactobacillus casei FPGS molecule showing the overall fold and secondary structural elements of L. casei FPGS (left panel). The van der Waals surface of L. casei FPGS (right panel). Folylpolyglutamate synthetase is responsible for the addition of a polyglutamate tail to folate and folate derivatives. It is an ATP-dependent enzyme isolated from eukaryotic and bacterial (prokaryotic) sources, where it plays a key role in the retention of the intracellular folate pool. The crystal structure of L. casei MgATP complex of the enzyme FPGS, determined at 2.4 Å resolution, reveals that FPGS is a modular protein consisting of two domains, one with a typical mononucleotide-binding fold and the other strikingly similar to the folate-binding enzyme dihydrofolate reductase (DHFR) [8]. [PDB ID: 1FGS]
The synthesis of folylpolyglutamates, in addition to factors that regulate this synthesis, plays a significant role in the regulation of not only folate homeostasis but also in the regulation of cellular pools of folate. Mammalian cells possess two types of enzymatic activities that can potentially and directly regulate this process:
- Folylpolyglutamate synthetase that catalyzes the synthesis of folylpoly-γ-glutamates (Figure 5).
- γ-Glutamyl hydrolase, a lysosomal peptidase that can hydrolyze the folate polypeptide chain (Figure 6).
Folylpolyglutamate synthetase is localized in the cytosol and mitochondria of mammalian tissues and cells. Moreover, the mitochondrial isozyme is required for the accumulation of normal mitochondrial folate pools. Nevertheless, folylpolyglutamates cannot be transported into the mitochondria, folylpolyglutamates synthesized in the mitochondria are gradually released into the cytosol (Figure 1).
4. Role of Intracellular Folate-Binding-Proteins:
Binding of folylpolyglutamates to various cellular proteins, primarily enzymes of one-carbon metabolism would be expected to reduce the availability of some of these compounds for other enzymes of one-carbon metabolism and would assist in folate retention by tissues.
Liver contains five major folate-binding proteins. A large proportion of hepatic mitochondrial THF polyglutamate is associated with sarcosine and dimethylglycine dehydrogenases [9]. The cytoplasmic folate-binding protein, glycine N-methyl transferase, is present in high concentrations and contains bound 5-methyl-THF polyglutamate, which is an inhibitor of the enzyme.
Many of the enzymes of one-carbon metabolism are present at much higher levels in liver than in other tissues. This possibly reflects their role in hepatic gluconeogenesis. Peripheral tissues have lower levels of both folate-binding proteins and cellular folate. Since protein binding limits the availability of folate substrates for various enzymes of one-carbon metabolism, it is not always intuitive how changes in folate availability influence folate and one-carbon metabolism. Suggesting that protein binding may partially buffer one-carbon metabolism over a wide range of physiological tissue folate concentrations, making one-carbon metabolism less responsive to changes in folate concentration.
5. Role of γ-Glutamyl Hydrolase (GGH):
Mammalian tissues contain GGH (conjugases) that catalyze the hydrolysis of folylpoly-γ-glutamates and antifolylpoly-γ-glutamates by the removal of γ-linked polyglutamates and glutamate. This enzyme hydrolyzes the polyglutamate sidechains of pteroylpolyglutamates. Progressively removes γ-glutamyl residues from pteroylpoly-γ-glutamate to yield pteroyl-α-glutamate (folic acid) and free glutamate. Thus, may play an important role in the bioavailability of dietary pteroylpolyglutamates and in the metabolism of pteroylpolyglutamates and antifolates (Figure 6).
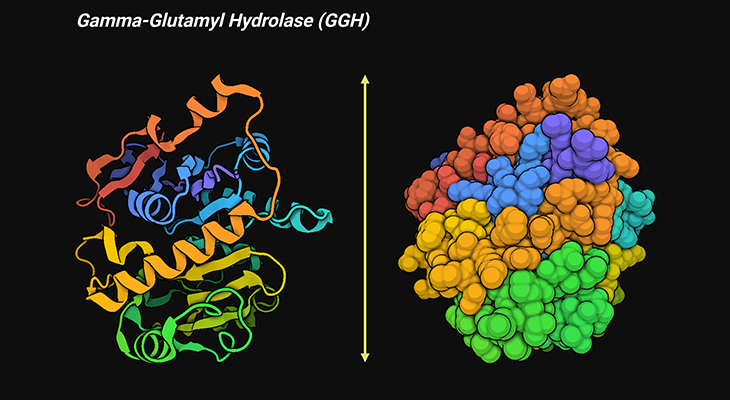
Figure 6. Three-Dimensional Structure of Human Gamma-Glutamyl Hydrolase (GGH). Ribbon diagram of a single human GGH molecule showing the overall fold and secondary structure elements of human GGH (left panel). The van der Waals surface of human GGH (right panel). γ-Glutamyl hydrolase catalyzes the cleave of the γ-glutamyl chain of folylpoly-γ-glutamyl substrates. It is a central enzyme in folyl and antifolyl poly-γ-glutamate metabolism. The crystal structure of human GGH, determined at 1.6 Å resolution, reveals that the protein is a homodimer. The overall structure of human GGH contains 11 α-helices and 14 β-strands, with a fold in which a central eight-stranded β-sheet is sandwiched by three and five α-helices on each side [10]. [PDB ID: 1L9X]
Longer-chain folylpolyglutamates are typically better substrates than the shorter glutamate chain length derivatives. In most tissues, GGHs are located in the cytoplasmic organelle lysosome, have an acid pH optimum, and appear to be mainly endopeptidases. Some plasma GGHs function at physiological pH. Since plasma contains GGH activity, any polyglutamate that is released into plasma would be hydrolyzed into the monoglutamate. The role of lysosomal GGH in folate homeostasis has not been firmly established but presumably involved, at a minimum, in catabolism of folate cleavage products.
Conclusions
In this blog, we have summarized our current perspectives of the mechanisms by which folates stored in tissues and in the body are regulated, i.e., an overview of folate homeostasis/tissue accumulation. During the past decade, considerable progress has been made in our understanding of not only the properties of various folate transporters responsible for folate influx into tissues, but also on the mechanisms by which folate is metabolized and retained in tissue, especially under physiological conditions. However, our understanding of potential defects in these processes under pathophysiological conditions is more restricted. In addition, the mechanisms governing folate turnover and excretion are still poorly understood, at present.
For information on autism monitoring, screening and testing please read our blog.
References
- Chandler CJ, Wang TT, Halsted CH. Pteroylpolyglutamate hydrolase from human jejunal brush borders. Purification and characterization. J Biol Chem. 1986 Jan 15;261(2):928-33. PMID: 2867095.
https://pubmed.ncbi.nlm.nih.gov/2867095/ - Zhao R, Matherly LH, Goldman ID. Membrane transporters and folate homeostasis: intestinal absorption and transport into systemic compartments and tissues. Expert Rev Mol Med. 2009 Jan 28;11:e4. doi: 10.1017/S1462399409000969. PMID: 19173758; PMCID: PMC3770294.
https://pubmed.ncbi.nlm.nih.gov/19173758/ - Williams WM, Huang KC. Renal tubular transport of folic acid and methotrexate in the monkey. Am J Physiol. 1982 May;242(5):F484-90. doi: 10.1152/ajprenal.1982.242.5.F484. PMID: 7081435.
https://pubmed.ncbi.nlm.nih.gov/7081435/ - Shane B. Folylpolyglutamate synthesis and role in the regulation of one-carbon metabolism. Vitam Horm. 1989;45:263-335. doi: 10.1016/s0083-6729(08)60397-0. PMID: 2688305.
https://pubmed.ncbi.nlm.nih.gov/2688305/ - Lin BF, Shane B. Expression of Escherichia coli folylpolyglutamate synthetase in the Chinese hamster ovary cell mitochondrion. J Biol Chem. 1994 Apr 1;269(13):9705-13. PMID: 8144561.
https://pubmed.ncbi.nlm.nih.gov/8144561/ - Lin BF, Huang RF, Shane B. Regulation of folate and one-carbon metabolism in mammalian cells. III. Role of mitochondrial folylpoly-gamma-glutamate synthetase. J Biol Chem. 1993 Oct 15;268(29):21674-9. PMID: 8408020.
https://pubmed.ncbi.nlm.nih.gov/8408020/ - Cook JD, Cichowicz DJ, George S, Lawler A, Shane B. Mammalian folylpoly-gamma-glutamate synthetase. 4. In vitro and in vivo metabolism of folates and analogues and regulation of folate homeostasis. Biochemistry. 1987 Jan 27;26(2):530-9. doi: 10.1021/bi00376a027. PMID: 3828323.
https://pubmed.ncbi.nlm.nih.gov/3828323/ - Sun X, Bognar AL, Baker EN, Smith CA. Structural homologies with ATP- and folate-binding enzymes in the crystal structure of folylpolyglutamate synthetase. Proc Natl Acad Sci U S A. 1998 Jun 9;95(12):6647-52. doi: 10.1073/pnas.95.12.6647. PMID: 9618466; PMCID: PMC22582.
https://pubmed.ncbi.nlm.nih.gov/9618466/ - Wittwer AJ, Wagner C. Identification of the folate-binding proteins of rat liver mitochondria as dimethylglycine dehydrogenase and sarcosine dehydrogenase. Purification and folate-binding characteristics. J Biol Chem. 1981 Apr 25;256(8):4102-8. PMID: 6163777.
https://pubmed.ncbi.nlm.nih.gov/6163777/ - Li H, Ryan TJ, Chave KJ, Van Roey P. Three-dimensional structure of human gamma -glutamyl hydrolase. A class I glatamine amidotransferase adapted for a complex substate. J Biol Chem. 2002 Jul 5;277(27):24522-9. doi: 10.1074/jbc.M202020200. Epub 2002 Apr 12. PMID: 11953431.
https://pubmed.ncbi.nlm.nih.gov/11953431/