Table of Contents
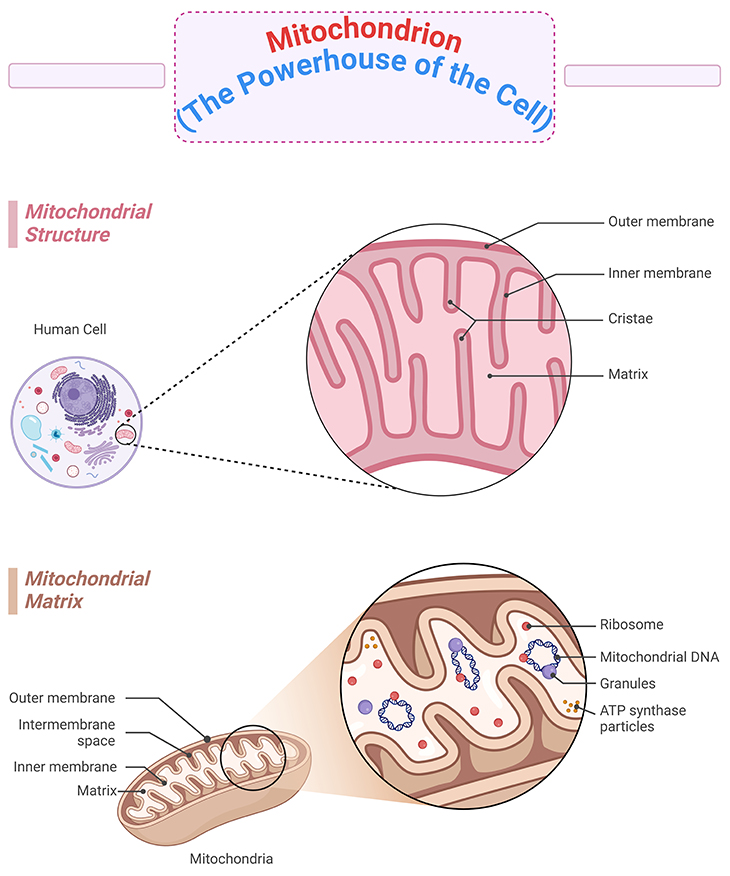
Figure 1. The powerhouse of the cell – the structure of the mitochondrion. Most cells have many mitochondria, which produce ATP as a form of cellular energy. The multifaceted roles of mitochondria in the central nervous system (CNS) – indeed, mitochondria are crucial for various cellular processes, especially in neurons where they support metabolism and calcium homeostasis. Their dynamic nature, including their ability to divide, fuse, and move, ensures they are well-distributed to meet the high energy demands of neurons. Recent advancements in imaging and genetic tools have significantly enhanced our understanding of mitochondrial functions. These techniques have revealed how mitochondria influence axon branching, synaptic function, calcium regulation with the endoplasmic reticulum (ER), glial cell function, neurogenesis, and neuronal repair. Mitochondrial dysfunction is linked to numerous CNS disorders, from impaired neuronal development to neurodegenerative diseases. Most importantly, understanding the role of mitochondria in ASD could lead to more effective diagnostic and therapeutic strategies, potentially improving the quality of life for those affected.
Introduction
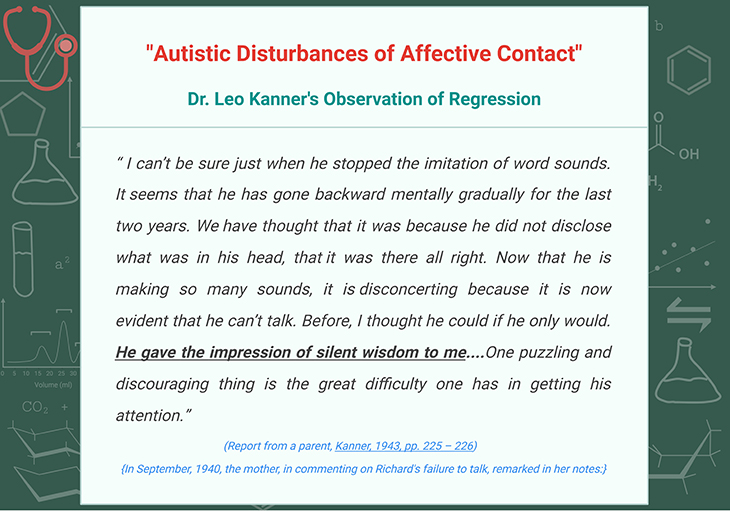
Box-1. Autistic disturbances of affective contact. Dr. Leo Kanner’s observation of regression, a case study. (Kanner, 1943, pp. 225 – 226) [1].
As the above quote from a parent illustrates observations of regression have been intricately linked with autism from the time of its designation in 1943, when Leo Kanner first published the case histories of eleven children with “autistic disturbances of affective contact” (see Box-1) [1].
What is Regression?
Regression in autism usually occurs within the second year of the life and can happen either abruptly or gradually. The child experiences a stall in skill acquisition or a measurable loss, which usually occurs in the areas of language and/or social skills, although there are occasional reports of the loss of adaptive and fine motor skills too. Eventually, the regressive period is succeeded by the regaining of skills, although some children may never fully regain the skills they had originally lost [2].
Anywhere between 10 – 50% of children who are diagnosed with autism have experienced regression, with the most common reported figures hovering around one third of cases. These estimates probably vary because of differences in criteria used to define “regression.”
Some studies for instance restrict it to extreme forms of language loss, while others include subtler plateaus in language and social skills. However, estimates also probably vary modestly because the primary means of estimating regression in autism is generally parental report. While parents know their children better than anyone else, especially in those early formative years, human memory is not infallible – particularly if the regressive losses are subtle and the parent is answering a questionnaire ten years after the fact.
Remarkably, regression appears to be largely unique to autism. When comparing autistic toddlers with other children with various intellectual disabilities, researchers have found that language regression occurs in a large minority of those with autism, however, it is rarely seen in those with intellectual disability alone [2].
Regression in Syndromic Forms of Autism
Regression also occurs in some syndromic forms of autism, such as Rett syndrome and childhood disintegrative disorder (CDD) (once known as Heller’s syndrome), both of which had previously been grouped under the DSM-IV’s autism umbrella, Pervasive Developmental Disorders, but have since been removed.
(i) Rett Syndrome
Most cases of Rett syndrome are linked with loss-of-function mutations involving the MECP2 gene, which produces a protein that binds to methylated DNA and helps to control gene expression. Almost all cases of Rett syndrome are female due to the lethality of the X-linked mutation in males. When it does occur in males, this is typically due to something known as somatic mosaicism, in which the mutation is not present in all cells of the body but only a portion because it has occurred later in embryonic development rather than in the originating germ cells.
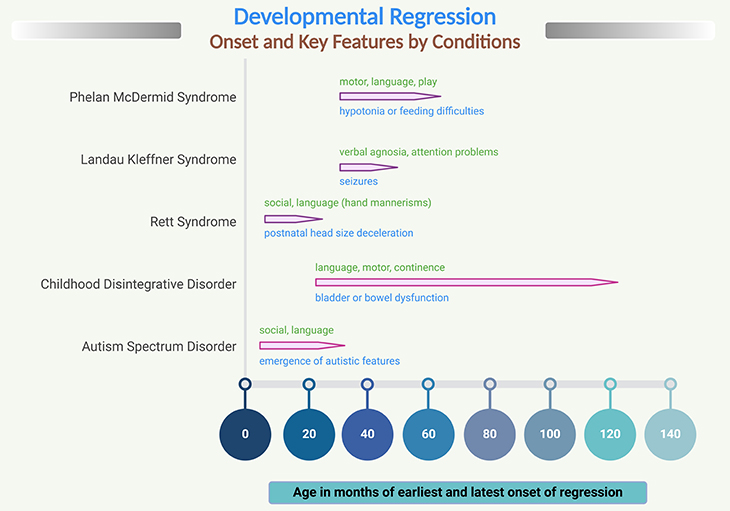
Figure 2. Developmental regression onset and key features by condition. Prevalence: autism 1:44 – 100; Rett syndrome 1:10,000 – 15,000; Childhood disintegrative disorder 1.4 – 9.2 per 100,000; Phelan McDermid syndrome 2 – 10 per 1000000; Landau Kleffner syndrome 1 per 1000000. (Green – regression of developmental skills. Blue – other typical features may be identified before onset of regression)
Prior to regression, the majority of girls with Rett’s present with some type of developmental delay. Regression onset then usually occurs between 12 to 18 months of age, with an average of 16 months, which is slightly younger than the average 20 months in idiopathic autism (see Figure 2) [2-3].
The most prominent feature in Rett syndrome is a decline in motor skills involving the hands, often leading to the characteristic “hand wringing” feature for which Rett’s is known. However, large numbers of girls also lose language, nonverbal communication and play, and other motor skills [3].
(ii) Childhood Disintegrative Disorder (CDD)
Unlike Rett syndrome, CDD is a poorly studied and understood condition that occurs more often in boys. Currently, there are no known mutations associated with CDD, which may reflect the state of our science but may also suggest that, like autism, it is a heterogeneous disorder. Occasional case studies reporting the occurrence of autism and CDD in the same families suggests the latter may also be true [4].
CDD is typified by normal or near-normal development until at least two years of age, after which a regression period begins that may be characterized by high levels of anxiety, followed by a severe decline in skills and the onset of autistic symptomology. Often, language is lost and rarely regained, as are self-help skills such as appropriate use of the toilet or feeding. Unfortunately, most children with CDD develop intellectual disability and are severely affected for the rest of their lives (see Figure 2).
What Causes Regression?
Some types of autism have variable hereditary pattern. For instance, parents of multiplex (multiple incidence) families often exhibit a broader autism phenotype (BAP) themselves. Meanwhile, parents who have only a single child with autism (simplex cases) are considerably less likely to have autistic features than multiplex parents.
Although, it may seem surprising given the typical developmental trajectory of children with autistic regression, typified by near-normal development followed by sudden or gradual regression, parents of these children have similar rates of BAP as parents of children without regression. This suggests that regressive autism, on the whole, is as heritable as non-regressive autism.
Over-Pruning Hypothesis of Autistic Regression
We know that regressive autism may be partly inherited, but what causes it? One of the more popular current theories is the over-pruning hypothesis of autistic regression [5]. According to this hypothesis, the loss of neural connectivity leading to regression is due to overactive pruning of those connections, which normally starts to occur in toddlerhood and extends throughout childhood. Curiously, the neurodevelopmental model used to test this hypothesis does seem to support the idea that regression is due to a loss of connectivity – however, it never proves what causes that loss (e.g., pruning), merely that there is indeed a loss. (Cf. previous blogs entitled as: “The Neurotypical Brain versus the Autistic Brain – Neurotypical Brain Development and Function” & “The Neurotypical Brian versus the Autistic Brain: Brain Development and Function in ASD”).
In addition, the brain in autism is not typified by generalized underconnectivity and in fact has a wealth of short-range connective fibers. Instead, the larger longer fibers of the brain are preferentially depleted.
Many cases of autistic regression seem to be linked with some kind of stressor or stimulus as reviewed in the following sections in this blog and subsequent ones. Sometimes, that stressor is the onset of an epileptic disorder. Other times, that stimulus is an illness. Sometimes, it is both. Consequently, it implies that regression is the result of excessive or chronic cellular stress, leading to the rapid or slow loss of synapses, neural connections, and sometimes cells themselves.
This loss leads to a subsequent loss in skills, such as language and socialization. Sometimes these skills may be regained, especially in smaller neural networks in which regrowth is possible. However, long-range connections are unlikely ever to be recovered owing to the need for specific developmental cues that lead connections along their arduous paths and that are no longer present in the postnatal brain. In this way, coordination amongst disparate functional modules of the brain will be permanently impaired.
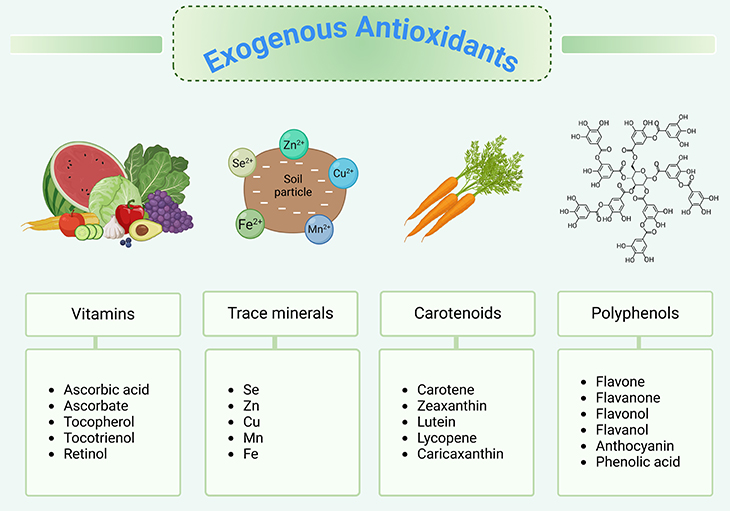
Figure 3. Exogenous antioxidants. In general, a plant-based diet protects against chronic oxidative stress-related diseases. Dietary plants contain variable chemical families and amounts of antioxidants. It has been hypothesized that plant antioxidants may contribute to the beneficial health effects of dietary plants.
Oxidation
(i) Free Radicals and Antioxidants
Everyone has probably heard the term, antioxidant, in the context of nutrition. We know that many plant-based foods, such as berries or spices like turmeric, tend to be very high in antioxidants. Meanwhile, animal-based foods such as eggs or fish are generally fairly low (see Figure 3) [6].
Antioxidants help to neutralize free radicals, which are molecules that have unpaired electrons, a state that leaves them chemically unstable (see Figure 4). In the case of biological systems, we are usually most interested in oxygen-based free radicals known as reactive oxygen species (ROS), because they are a common metabolic byproduct (see Figure 5).
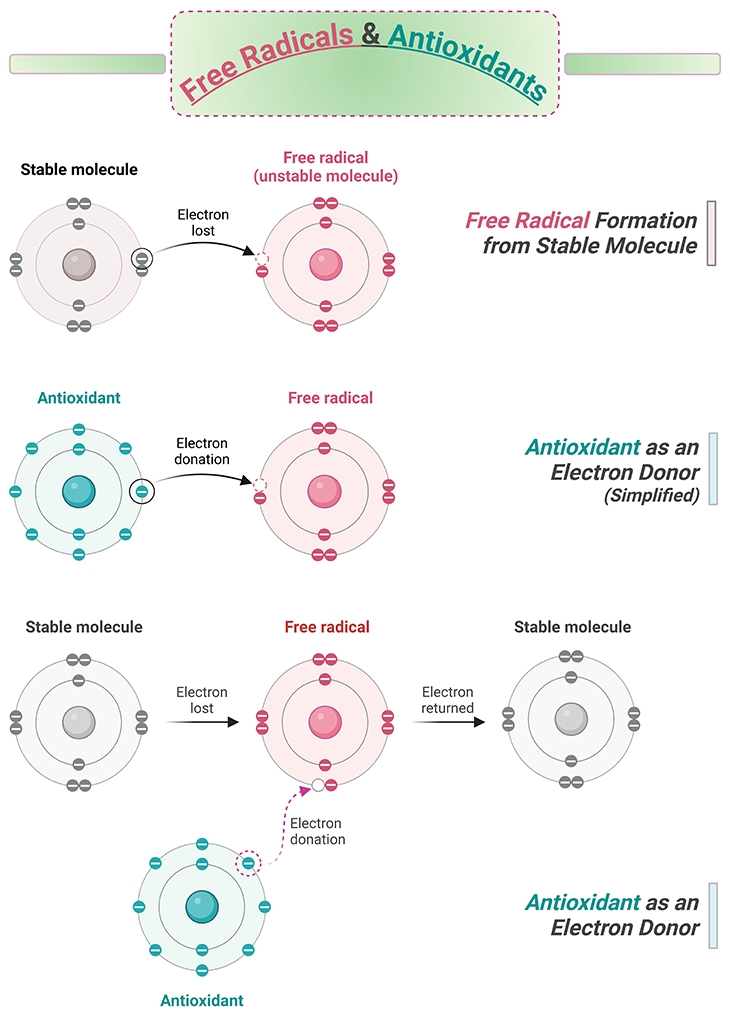
Figure 4. Free radicals and antioxidants. A free radical, known for stealing electrons from other molecules thereby creating a chain reaction of free radicals. Antioxidants help to neutralize free radicals.
In order to stabilize, free radicals steal electrons from other molecules around them. However, in doing so, whatever molecule has been robbed of its electron typically becomes a free radical itself. This can lead to a long chain of electron thievery, leaving misshapen and poorly functioning molecules in its wake (see Figure 4).
Fortunately, the body has a supply of antioxidants that shuts down this runaway reaction, either by donating electrons or degrading the free radical itself. As we mentioned, any of the antioxidants in our bodies are derived from the foods we eat, most of which come from fruits and vegetables and include the vitamins, A, C, and E. However, we also produce antioxidants ourselves, such as glutathione (see Figure 3-4; Figure 6).
In fact, the sleep hormone, melatonin, is a very powerful antioxidant. Melatonin is mostly produced by the brain’s pineal gland, beginning in the evening hours and reaching its peak at night. Melatonin production is suppressed by exposure to the blue end of the light spectrum, which is why sleep specialists recombined the use of dim yellow lighting in the evenings to mimic effects of the setting sun.
(ii) Mitochondrial Dysfunction and Reactive Oxygen (ROS)
Mitochondria are the powerhouses of the cell. Through complex chain of events known as respiration, mitochondria take energy from food we eat (e.g., glucose) and the air we breathe (e.g., oxygen) and produce the basic energy currency of the cell, adenosine triphosphate (ATP). The cell can the take that ATP and break off phosphate molecules to produce an energy catalyst for processes within the cell (see Figure 1).
ROS are major byproducts of cellular respiration. Whenever the cell is overrun by ROS, this leads to oxidative stress, which in turn triggers a host of reactions termed the “oxidative response.” In bacteria, this response is fairly straightforward because they tend to live in environments with fluctuating oxygen levels, therefore the presence of ROS can trigger an oxidative response.
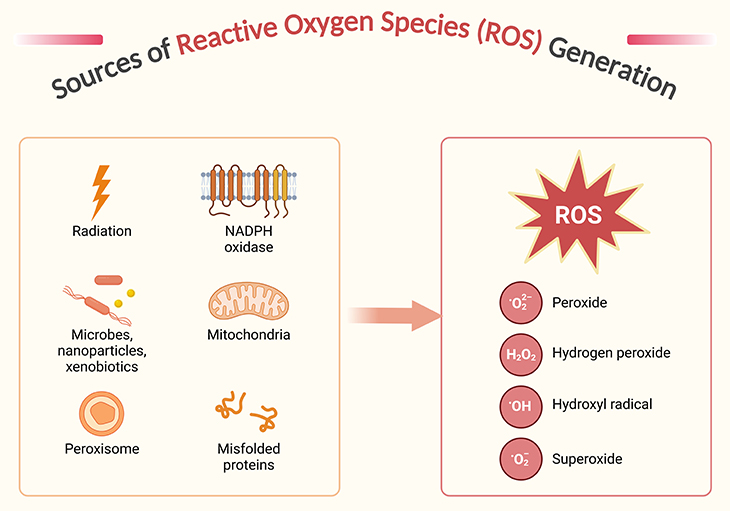
Figure 5. Sources of reactive oxygen species generation. Reactive oxygen species (ROS) and their reactivity – indeed, while oxygen is vital for life, its derivatives, particularly ROS, can be quite harmful when present in excess. ROS are a diverse group of molecules, including both free radicals (like superoxide anion, O2•−, and hydroxyl radical, •OH) and non-radicals (like hydrogen peroxide, H2O2). Free radicals are particularly reactive due to their unpaired electrons, which drive them to “steal” electrons from other molecules, initiating a chain reaction of molecular damage. The production of ROS occurs through various cellular processes, including the one-, two-, and three-electron reductions of molecular oxygen, leading to the formation of superoxide, hydrogen peroxide, and hydroxyl radicals, respectively. While superoxide and hydroxyl radicals are highly reactive, hydrogen peroxide is less so but can still contribute to oxidative stress. Cells have evolved sophisticated antioxidant defenses to manage ROS levels and mitigate their potential damage. These defenses include enzymatic antioxidants like superoxide dismutase and catalase, as well as non-enzymatic antioxidants like vitamins C and E6.
Eukaryotic organisms such as ourselves, however, live in environments in which oxygen levels are indicator of impending damage but a normal part of respiration. Thus, it is the quantity of ROS, not their presence, which leads to a complicated cascade of events in mammalian cells that help not only to deal with increased ROS load but also repair damage to cellular structures. In some cases, when extreme, these processes may even lead to apoptosis or cell suicide [7].
(iii) How Oxidative Stress Damages the Brain
Because we need oxygen to survive, our cells are constantly bombarded by ROS, leading to perpetual damage of DNA, proteins, and fats that must constantly be repaired (see Figure 5). However, ROS are not only byproducts of cellular metabolism, but they also play important roles in various molecular pathways, such as inflammation, and therefore we are always in a precarious state of balance between having too much and too little ROS. Therefore, it is important to eat antioxidant-rich foods but also not to take excessive amounts of antioxidant supplements because we need some ROS for our cells to work properly (see Figure 6) [8].
Unfortunately, as we age the ability of our tissues to repair themselves following ROS damage declines. The brain is especially vulnerable to oxidative damage because of its high oxygen demands requiring about 20% of the body’s basal consumption. In addition, many things can go wrong in the brain, leading either to increases in ROS or impairments in the mechanism that deal with them.
For example, excessive availability of the excitatory neurotransmitter glutamate can lead to cell damage or death through “excitotoxicity” or the excessive stimulation of neurons. Oxidative stress can damage neurons, leading to a release of glutamate into the surrounding space, triggering an excitotoxic domino effect. This excitotoxic storm is one of the major risks following stroke, as cellular damage from the initial ischemic attach release excessive glutamate into the extracellular space, overstimulating intact neurons surrounding the original lesion. Consequently, stroke patients need to be treated rapidly with medications that prevent the spread of excitotoxicity to surround areas of the brain [9].
Metal ions, such as iron, can also act as free radicals and are released into the extracellular space following neuronal damage. Unfortunately, because the brain has little iron-binding capacity, the presence of these metal ions can persist for long periods of time, triggering oxidative stress until they are finally flushed from the cerebral spinal fluid (CSF).
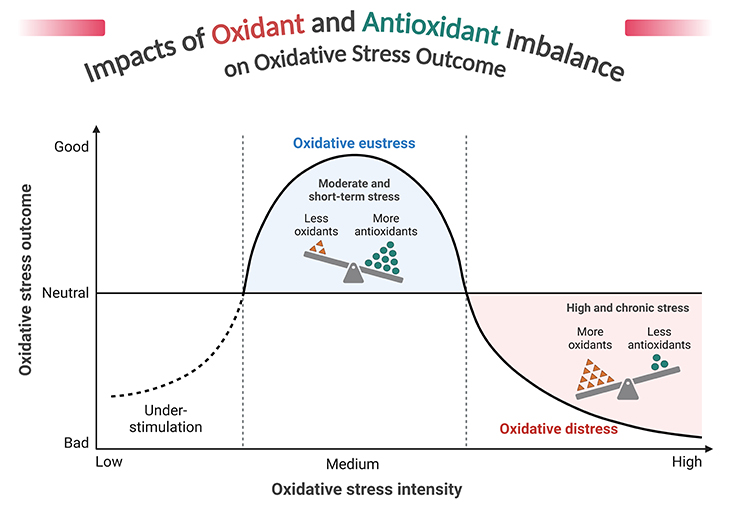
Figure 6. Impacts of oxidant and antioxidant imbalance on oxidative stress outcome. Reactive oxygen species (ROS) and oxidative stress – indeed, ROS are a double-edged sword in cellular biology. While they play crucial roles in cell signaling and homeostasis, their overproduction can lead to oxidative stress, causing significant damage to cellular components like DNA, proteins, and lipids. Oxidative stress is implicated in the pathogenesis of numerous diseases, including cancer, cardiovascular diseases, diabetes, and neurodegenerative disorders such as Alzheimer’s and Parkinson’s. The body employs a variety of antioxidant defenses, both enzymatic (e.g., superoxide dismutase, catalase) and non-enzymatic (e.g., vitamins C and E, glutathione), to counteract the harmful effects of ROS. The balance between ROS production and antioxidant defenses is crucial. When this balance tips in favor of ROS, oxidative stress occurs, leading to cellular damage and contributing to aging and various diseases. Cells have evolved numerous stress response mechanisms to manage oxidative stress, including pathways that promote cell survival or, in some cases, cell death, depending on the context.
The fatty membranes of neurons are also common targets of oxidation in a process known as “lipid peroxidation.” When lipid peroxidation occurs in excess, the membranes of cells can become more permeable, allowing in toxic agents that damage the cell.
The functions of ion channels and receptors within the membrane can also be irreparably damaged, ultimately leading to cell death and many of the processes mentioned above (e.g., release of glutamate and metal ions into the surround tissue).
In contrast to much of the rest of the body, antioxidant defense is comparatively modest in the brain. Considering the communicative roles some ROS play in brain function, such as nitric oxide, this may well be an adaptive evolutionary strategy. However, with such benefits come risks, notably increased oxidative stress over time.
With time also come mutations. Not only do we house DNA within the nucleus of our cells, but our mitochondria also contain their own small genomes, which, like nuclear DNA, acquire more and more mutations over time that affect the mitochondria’s capacity to function properly. Because mitochondria produce a lot of ROS, many of these mutations are like the result of oxidative damage. Impaired mitochondrial function can lead to excessive ROS production and further oxidative stress, resulting in a positive feedback loop (see Figure 5).
Finally, inflammation also drives oxidative stress. Astrocytes, a type of glial (helper) cell, produce inflammatory molecules such as various cytokines. These cytokines then stimulate immune cells within the brain known as microglia. These microglia release more inflammatory molecules, as well as various ROS, as part of their inflammatory response [8; 10]. If this type of reaction occurs chronically, it can lead to progressive damage as seen in some types of neurodegenerative disorders.
There are many more ways in which ROS can damage the cell. However, the majority of these mechanism feed into one another, leading or progressive damage regardless of which pathway is stimulated first. All of these processes together lead to aging of the brain and, in those vulnerable individuals, play pivotal roles in the development of senile neurodegeneration. However, as we will discuss in the next section, they may play important roles in neurodevelopmental conditions such as autism as well.
(iv) Mitochondrial Diseases and Dysfunction in Autism
More than three decades ago, researchers realized that the mitochondrion, the powerhouse of cell (see Figure 1), may play a role in autism susceptibility. We now know that mitochondrial disorders occurs in roughly 7% of the autism spectrum population, making it one the most common “rare” disorders associated with the condition.
However, the vast majority of autistic people may have some kind of mitochondrial dysfunction, although in most cases it may be a secondary symptom of another underlying problem, such as chronic inflammation [11]. It is likely, however, that mitochondria play an integral role in positive feedback loops that main this chronicity.
Individuals with certain types of mitochondrial disease are sometimes vulnerable to immunological challenges, such as illness and vaccination, which can trigger cognitive decline, a feature reminiscent of regression in autism [12]. Remarkably, about 60% of children with both autism and mitochondrial disease experience regression, rates that are twice as high as those seen in the rest of the spectrum [13; 14].
What is more, the vast majority (~70%) of these children regress following a fever-related illness or vaccination. However, vaccination does not appear to trigger regression in the absence of fever, suggesting the extent of immunological challenge is the driving factor in disease onset in these cases [13].
Although true mitochondrial disorders occur in only a small minority of those with autism, genetics research suggests that many autistic children have mitochondrial mutation profiles similar to those seen in older people, indicating oxidative damage. Curiously, the same patterns of oxidative damage are seen in mothers of children with autism, suggesting that damage to the DNA may occur maternally and is then inherited by the child [15].
Because of the mitochondrial DNA’s proximity to the production of ROS within the mitochondrion, it is more vulnerable to the effects of free radicals than nuclear DNA. And because we all inherit our mother’s mitochondria, we preferentially inherit our mothers’ mitochondrial mutations. Fortunately, women seem to produce far more antioxidants and fewer ROS than males, leading to a four-fold decrease in the comparative rates of mutation, an effect that is largely mediated by the hormone estrogen.
Undoubtedly, evolution has taken great strides to protect the mitochondria from harmful mutations in women because of their carrier status, whereas men are effectively mitochondrial dead ends. Unfortunately, this also means that males are more vulnerable to the effects of oxidative damage and mitochondrial dysfunction, which has been suggested as a reason why men on average do not live as long as women. In addition, if mitochondrial dysfunction plays a role in autism susceptibility, it may also help explain the higher prevalence in males.
Take Home Messages
What is Regression?
- Regression in Autism Spectrum Disorder (ASD) is a phenomenon where children who initially develop typically for the first one to two years of life experience an abrupt or gradual loss of previously acquired skills, particularly in language and social communication. This has been a topic of interest since the 1960s when reports of behavioral regression in children with autism began to emerge.
Prevalence and Characteristics
- Prevalence: A meta-analytic review of 85 studies involving 29,035 participants found that the overall prevalence of regression in ASD is 32.1%, with the mean age of onset being 1.78 years.
- Characteristics: The Autism Diagnostic Interview-Revised (ADI-R) is commonly used to characterize regression, requiring a loss of communicative use of at least five different words for at least three months.
Causes and Mechanisms
Despite extensive research, the exact causes and underlying neurobiological mechanisms of regression in ASD remain unclear:
- Vaccinations and Gastrointestinal Issues: Studies have explored associations with the MMR vaccine, gastrointestinal problems, and mitochondrial diseases, but none have identified significant risk factors.
- Traditional Risk Factors: Pre- and post-natal complications and viral infections have also been investigated without yielding conclusive causes.
- Epilepsy: Some studies found a high proportion of children with regression and comorbid epilepsy, but others did not find significant differences in epilepsy risk between regressive and non-regressive ASD.
- Genetic Factors: Research has not identified specific genetic differences between children who regress and those who do not.
Neurobiological Theories
- Neuroimaging: Various neuroimaging studies have been conducted, but none have consistently explained regressive ASD.
- Computer Models: Emerging computer-modeled theories are being explored but remain unproven.
Subtypes and Prognosis
- Regression Subtype: Prospective studies have shown that around 50% of children later diagnosed with ASD exhibit typical development before experiencing developmental changes, including regression. However, the full range of regression patterns is not yet fully understood.
- Prognosis: The long-term outcomes for children with regressive ASD compared to those without regression are mixed, with some studies reporting no significant differences.
Summary and Conclusions
Developmental regression in ASD indeed remains a complex and not fully understood phenomenon. Approximately one-third of children with ASD experience developmental regression, typically losing skills such as speech, nonverbal communication, social, or play skills during the preschool years. Many children who undergo regression often show subtle developmental differences before the regression occurs.
Predicting outcomes for children with ASD who experience regression is challenging. Early development of social, language, and attachment behaviors followed by regression does not necessarily predict better recovery or developmental outcomes.
The exact mechanisms behind developmental regression in ASD are still unknown. There is ongoing research into various potential factors, including genetic, neurological, and environmental influences. The role of subclinical epilepsy in developmental regression in children with autism is still unclear and remains a topic of investigation.
Understanding and addressing developmental regression in ASD requires further research to uncover the underlying causes and to develop effective interventions.
For information on autism monitoring, screening and testing please read our blog.
References
- Kanner L. Autistic disturbances of affective contact. Nervous Child. 1943 2:217-250.
https://www.autismtruths.org/pdf/Autistic%20Disturbances%20of%20Affective%20Contact%20-%20Leo%20Kanner.pdf - Baird G, Charman T, Pickles A, Chandler S, Loucas T, Meldrum D, Carcani-Rathwell I, Serkana D, Simonoff E. Regression, developmental trajectory and associated problems in disorders in the autism spectrum: the SNAP study. J Autism Dev Disord. 2008 Nov;38(10):1827-36. doi: 10.1007/s10803-008-0571-9. Epub 2008 May 1. PMID: 18449635.
https://pubmed.ncbi.nlm.nih.gov/18449635/ - Millichap JG. Patterns of regression in Rett syndrome. Pediatric Neurology Briefs. 2002 16:76-77.
https://pediatricneurologybriefs.com/articles/1573/files/submission/proof/1573-1-3203-1-10-20160313.pdf
- Zwaigenbaum L, Szatmari P, Mahoney W, Bryson S, Bartolucci G, MacLean J. High functioning autism and Childhood Disintegrative Disorder in half brothers. J Autism Dev Disord. 2000 Apr;30(2):121-6. doi: 10.1023/a:1005455505211. PMID: 10832776.
https://pubmed.ncbi.nlm.nih.gov/10832776/ - Thomas MS, Davis R, Karmiloff-Smith A, Knowland VC, Charman T. The over-pruning hypothesis of autism. Dev Sci. 2016 Mar;19(2):284-305. doi: 10.1111/desc.12303. Epub 2015 Apr 6. PMID: 25845529.
https://pubmed.ncbi.nlm.nih.gov/25845529/ - Carlsen MH, Halvorsen BL, Holte K, Bøhn SK, Dragland S, Sampson L, Willey C, Senoo H, Umezono Y, Sanada C, Barikmo I, Berhe N, Willett WC, Phillips KM, Jacobs DR Jr, Blomhoff R. The total antioxidant content of more than 3100 foods, beverages, spices, herbs and supplements used worldwide. Nutr J. 2010 Jan 22;9:3. doi: 10.1186/1475-2891-9-3. PMID: 20096093; PMCID: PMC2841576.
https://pubmed.ncbi.nlm.nih.gov/20096093/ - Martindale JL, Holbrook NJ. Cellular response to oxidative stress: signaling for suicide and survival. J Cell Physiol. 2002 Jul;192(1):1-15. doi: 10.1002/jcp.10119. PMID: 12115731.
https://pubmed.ncbi.nlm.nih.gov/12115731/ - Halliwell B. Oxidative stress and neurodegeneration: where are we now? J Neurochem. 2006 Jun;97(6):1634-58. doi: 10.1111/j.1471-4159.2006.03907.x. PMID: 16805774.
https://pubmed.ncbi.nlm.nih.gov/16805774/ - Hazell AS. Excitotoxic mechanisms in stroke: an update of concepts and treatment strategies. Neurochem Int. 2007 Jun;50(7-8):941-53. doi: 10.1016/j.neuint.2007.04.026. Epub 2007 May 10. PMID: 17576023.
https://pubmed.ncbi.nlm.nih.gov/17576023/ - Duncan AJ, Heales SJ. Nitric oxide and neurological disorders. Mol Aspects Med. 2005 Feb-Apr;26(1-2):67-96. doi: 10.1016/j.mam.2004.09.004. Epub 2005 Jan 24. PMID: 15722115.
https://pubmed.ncbi.nlm.nih.gov/15722115/
- Giulivi C, Zhang YF, Omanska-Klusek A, Ross-Inta C, Wong S, Hertz-Picciotto I, Tassone F, Pessah IN. Mitochondrial dysfunction in autism. JAMA. 2010 Dec 1;304(21):2389-96. doi: 10.1001/jama.2010.1706. PMID: 21119085; PMCID: PMC3915058.
https://pubmed.ncbi.nlm.nih.gov/21119085/
- Verity CM, Winstone AM, Stellitano L, Krishnakumar D, Will R, McFarland R. The clinical presentation of mitochondrial diseases in children with progressive intellectual and neurological deterioration: a national, prospective, population-based study. Dev Med Child Neurol. 2010 May;52(5):434-40. doi: 10.1111/j.1469-8749.2009.03463.x. Epub 2009 Sep 12. PMID: 19747204.
https://pubmed.ncbi.nlm.nih.gov/19747204/
- Shoffner J, Hyams L, Langley GN, Cossette S, Mylacraine L, Dale J, Ollis L, Kuoch S, Bennett K, Aliberti A, Hyland K. Fever plus mitochondrial disease could be risk factors for autistic regression. J Child Neurol. 2010 Apr;25(4):429-34. doi: 10.1177/0883073809342128. Epub 2009 Sep 22. PMID: 19773461.
https://pubmed.ncbi.nlm.nih.gov/19773461/ - Weissman JR, Kelley RI, Bauman ML, Cohen BH, Murray KF, Mitchell RL, Kern RL, Natowicz MR. Mitochondrial disease in autism spectrum disorder patients: a cohort analysis. PLoS One. 2008;3(11):e3815. doi: 10.1371/journal.pone.0003815. Epub 2008 Nov 26. PMID: 19043581; PMCID: PMC2584230.
https://pubmed.ncbi.nlm.nih.gov/19043581/ - Napoli E, Wong S, Giulivi C. Evidence of reactive oxygen species-mediated damage to mitochondrial DNA in children with typical autism. Mol Autism. 2013 Jan 25;4(1):2. doi: 10.1186/2040-2392-4-2. PMID: 23347615; PMCID: PMC3570390.
https://pubmed.ncbi.nlm.nih.gov/23347615/