Table of Contents
- Introduction
- Ramón y Cajal: The Architect of Neuronal Theory and Artistic Pioneer in Neuroscience
- Decoding the Connectome: Unraveling the Brain’s Intricate Network
- Neurotransmitter Symphony: The Molecular Dance of Neuronal Communication
- Synaptopathies
- Synaptopathies and Neurodevelopmental/Neuropsychiatric Disorders
- Spinogenesis
- Spinogenesis and Neurodevelopmental/Neuropsychiatric Disorders
- Take Home Messages
- Summary and Conclusions
- Did You Know About Folate Receptor Autoantibodies (FRAAs) and Brain Development?
- References
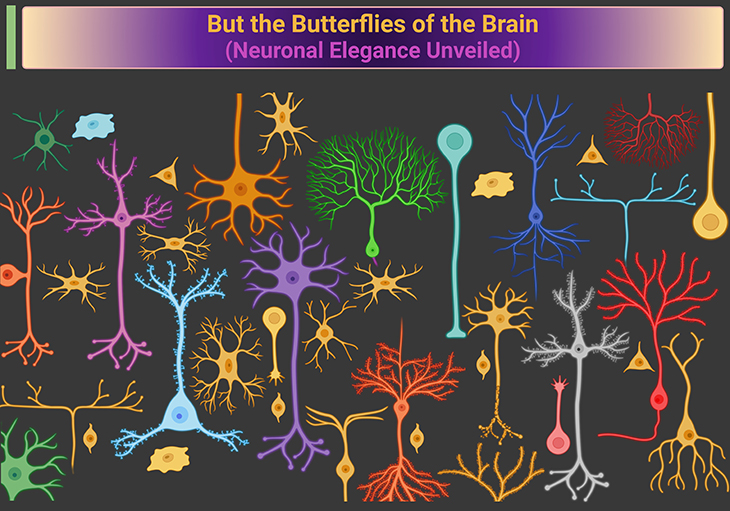
Figure 1. But the butterflies of the brain – Neuronal elegance unveiled. Just as butterflies flit gracefully through the air, neurons with their intricate, branching structures (dendritic trees) delicately connect and transmit life’s vital messages. Neural plasticity is the foundation of learning, where neurons continually adapt by growing, forming new branches, and creating or retracting synapses. This dynamic process of growth and pruning allows the brain to remodel itself based on experiences and learning, making it a marvel of adaptability and function. [Components of a neuron: All neurons contain three basic parts: a cell body and fibrous appendages called dendrites and axons.]
Introduction
Neural plasticity is the brain’s remarkable ability to adapt and reorganize itself throughout life. This involves the strengthening, weakening, formation, and elimination of synapses based on experiences and learning. Ramón y Cajal’s discovery of distinct neurons laid the groundwork for understanding neural plasticity. He recognized that neurons could grow, their branches could multiply, and new synapses could form or retract, a concept fundamental to the brain’s adaptive capabilities. Efforts to map the brain’s connectome, like those at the Allen Institute, are crucial for understanding neural plasticity. By revealing how neurons are interconnected and how these connections change over time, scientists can better understand the mechanisms underlying learning and memory.
Neurotransmitter exchange is essential for synaptic communication, which is central to neural plasticity. The dynamic process of neurotransmitter release and receptor binding enables synapses to strengthen or weaken, facilitating the brain’s ability to adapt and learn. Synaptic dysfunction (synaptopathies) can impair neural plasticity, contributing to neurodevelopmental and neuropsychiatric conditions like autism spectrum disorder (ASD) and schizophrenia. Understanding and addressing these synaptic abnormalities are vital for promoting healthy brain function and plasticity. Spinogenesis, the development of dendritic spines, is critical for synaptic connectivity and plasticity. Abnormalities in this process can disrupt neural plasticity, affecting cognitive and behavioral functions in neurodevelopmental and neuropsychiatric disorders.
These connections underscore the importance of understanding neural plasticity for advancing neuroscience and developing effective treatments for various brain disorders. The brain’s ability to reorganize itself is at the heart of learning, memory, and adaptation, making it a central theme in our exploration of the brain’s intricate workings.
Ramón y Cajal: The Architect of Neuronal Theory and Artistic Pioneer in Neuroscience
Santiago Ramón y Cajal, guided by his physician father, became fascinated with the microscopic study of the brain. Using the newly developed silver staining technique, which he refined, Cajal made groundbreaking discoveries about the brain’s cellular organization. This method, invented by Camillo Golgi, allowed Cajal to see the complete arborescence of individual nerve cells, leading to his refutation of Golgi’s reticular theory, i.e., nervous system formed a single ‘syncytium,’ a continuous net without any cellular boundaries. Cajal’s observations supported the ‘neuronal theory,’ establishing that the brain comprises distinct cells, or neurons, separated by gaps at synapses (see Figure 1; Figure 2) [1 – 2].
Cajal’s meticulous studies revealed how neurons transmit messages. He depicted nervous impulses originating from the cell body, traveling down the axon, and crossing synapses via neurotransmitters. He also recognized the plasticity of neurons, understanding that they could grow and form new synapses, a process now known to be fundamental to learning.
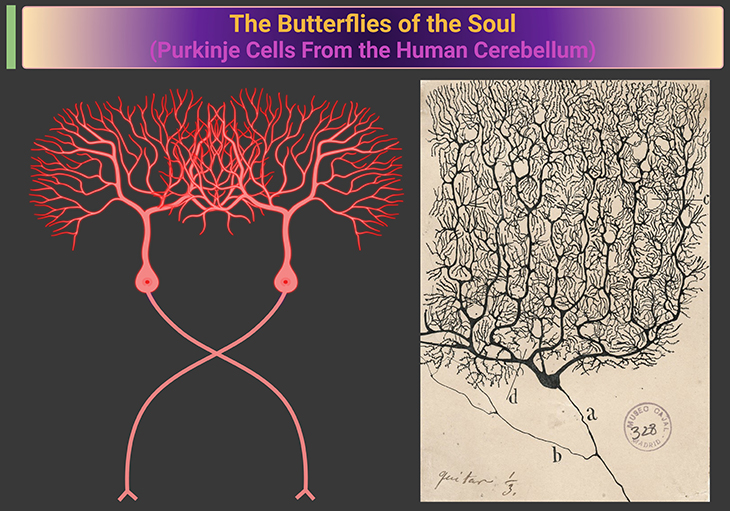
Figure 2. Neurons – The butterflies of the soul. Drawings of a neuron by Santiago Ramón y Cajal – Purkinje cell from the human cerebellum (Left, schematic drawing; right, original drawing). Purkinje cells, unique to the cerebellar cortex, are characterized by their large, flask-shaped cell bodies and extensive, flat, branched dendritic trees. These dendritic trees allow Purkinje cells to integrate a vast amount of information, playing a crucial role in motor coordination and cognitive functions. The cells’ single, long axon projects to the deep cerebellar nuclei, facilitating communication within the cerebellar circuitry. [Reticular versus neuron theory: In the late nineteenth century, scientists fiercely debated the question of whether the brain was made up of a reticulum of continuously connected elements or, instead, of individual cells, neurons, that communicated with one another. By the beginning of the twentieth century, the so-called neuron doctrine had emerged as the prevailing view.] {Image credit: Category: Purkinje cells – Wikimedia Commons}
In 1906, Ramón y Cajal and Golgi were jointly awarded the Nobel Prize in Physiology or Medicine for their contributions to understanding the nervous system. Cajal’s artistic anatomical drawings continue to inspire neuroscientists, highlighting the intricate beauty of neurons, which he lovingly called the “butterflies of the soul.”
Decoding the Connectome: Unraveling the Brain's Intricate Network
The function of a neuron is determined by its connections rather than its shape or location. Each cortical neuron forms about 10,000 synapses, which are essential for transmitting messages from other neurons. These synapses enable neurons to respond specifically as visual, auditory, tactile, motor, or cognitive cells.
To truly understand brain function, it’s crucial to map its complete connectivity matrix, identifying which neurons interact and the strength of their interactions. However, the human cortex contains roughly 86 billion neurons (about 1011 neurons), leading to a complex network of millions of billions of synapses (about 1015), intricately interwoven at a microscopic level.
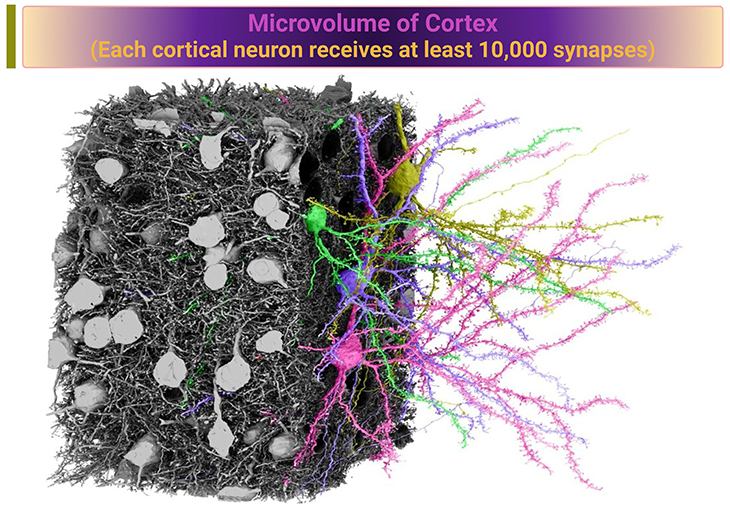
Figure 2. Microvolume of cortex – Each cortical neuron receives at least 10,000 synapses. Electron microscopy reconstruction of a 250 x 140 x 90 micron volume of mouse cortex. {Details and data available at https://microns-explorer.org – Image credit}
The Allen Institute in Seattle, funded by philanthropist Paul Allen, aims to provide a comprehensive description of the human brain, including its ‘connectome.’ This involves creating a detailed map of neuron types and their connections. Engineers there have developed automated electron microscopes that can capture nanometric images of cortical blocks.
As illustrated here in figure 2 and figure 3, in a small cortical volume containing 364 excitatory neurons, scientists in the MICrONS consortium have successfully reconstructed their dendrites, axons, and nearly all synapses using advanced algorithms [3].
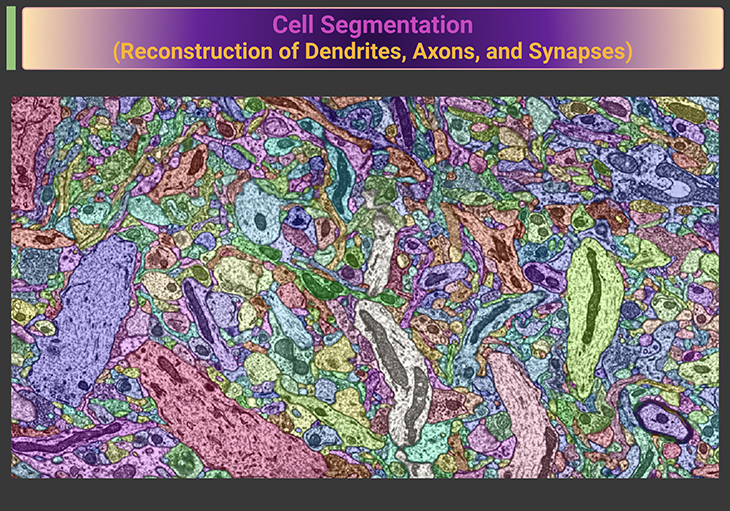
Figure 3. Cell segmentation – Reconstruction of dendrites, axons, and synapses. {Details and data available at https://microns-explorer.org – Image credit}
Future goals include extending this mapping to the entire brain in mice and humans and measuring neuronal activity in response to various stimuli. This effort seeks to determine if brain structure can predict function by understanding single neurons’ connectivity and responses. What an ambitious and fascinating project, wouldn’t you say?
Neurotransmitter Symphony: The Molecular Dance of Neuronal Communication
To truly understand the nervous system, it’s essential to examine it at the molecular level. Neurons communicate using chemical messengers called neurotransmitters—like acetylcholine, serotonin, dopamine, and glutamate.
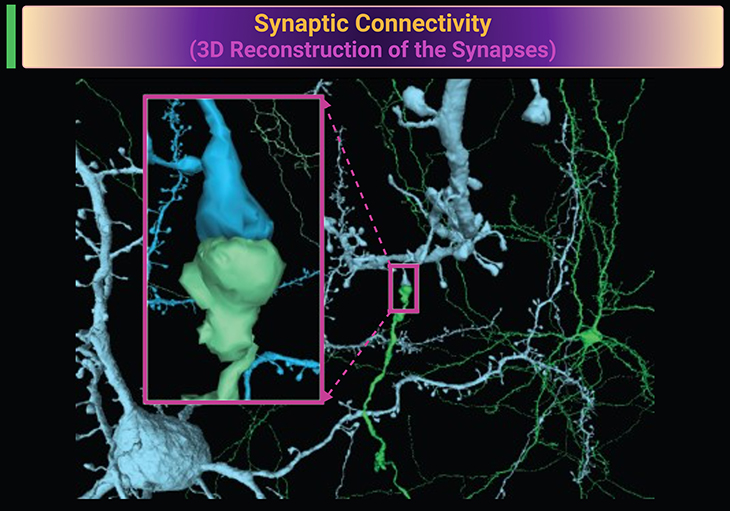
Figure 4. Synaptic connectivity. Three-dimensional (3D) reconstruction of the synapses that connect the axon of one neuron (green) with the dendrites of another neuron (blue). Reconstruction based on electron microscopy. Insert: showing the magnified view of a synaptic connectivity. Synapses are small gaps between neurons. When a neuron is active, an electrical impulse travels down its nerve fiber and causes the release of a chemical neurotransmitter from its terminal. The transmitter drifts across the synaptic space and binds to a dendrite on the receiving neuron, thus closing the gap. Essentially everything the brain does is accomplished by the process of synaptic transmission. {Details and data available at https://microns-explorer.org – Image credit}
These neurotransmitters are stored in vesicles within the presynaptic terminal of the axon. When an electrical signal reaches these vesicles, they release their contents into the synaptic cleft. The neurotransmitters then diffuse across this space and impact the postsynaptic neuron by binding to receptor molecules, akin to a key fitting into a lock (see Figure 4). (Cf. previous blog entitled as: The Neurotypical Brain versus the Autistic Brain: Neurotypical Brain Chemistry).
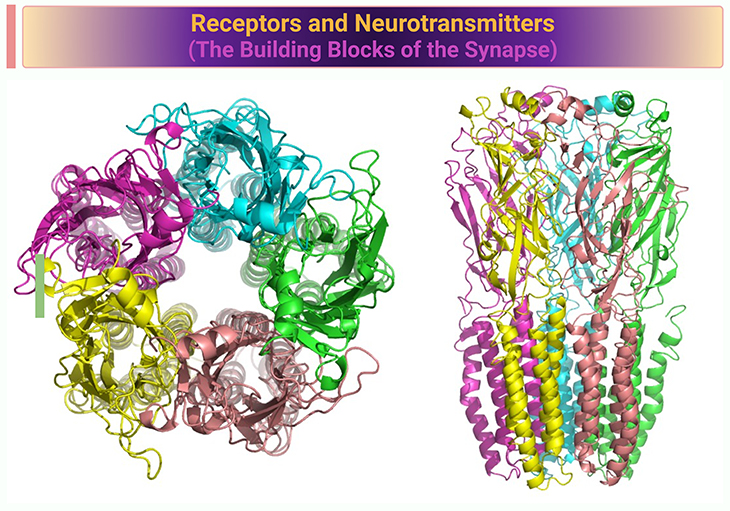
Figure 5. Receptors and neurotransmitters – The building blocks of the synapse. Three-dimensional (3D) reconstruction of the acetylcholine receptor. The acetylcholine receptor (AChR) is a pentameric protein complex, meaning it is composed of five subunits. These subunits are arranged around a central ion channel. The crystal structure of the AChR reveals several key features: (a) Cys-loop: The signature Cys-loop is a loop formed by a disulfide bond between two cysteine residues, which is crucial for the receptor’s function. (b) N-linked carbohydrate chains: These chains are attached to the extracellular domain of the receptor and play a role in its stability and function. (c) Main immunogenic region (MIR): This region is important for the immune response and is a target for antibodies. (d) Protein-protein and protein-sugar interactions: These interactions are essential for the binding of toxins and other molecules to the receptor. The crystal structure provides insights into how the receptor interacts with ligands and how it functions in synaptic transmission. This knowledge is crucial for understanding the molecular basis of diseases related to AChR dysfunction and for developing targeted therapies.{Image credit: Nicotinic acetylcholine receptor – Wikipedia}. [Kalamida et al. Muscle and neuronal nicotinic acetylcholine receptors. Structure, function and pathogenicity. FEBS J. 2007 Aug;274(15):3799-845. https://pubmed.ncbi.nlm.nih.gov/17651090/; Itier and Bertrand. Neuronal nicotinic receptors: from protein structure to function. FEBS Lett. 2001 Aug 31;504(3):118-25. https://pubmed.ncbi.nlm.nih.gov/11532443/]
Jean-Pierre Changeux, a French researcher, first isolated such a molecule, the acetylcholine receptor, in 1970 [4]. He utilized the fact that close variants of this receptor are found in the brain, in muscles, and in the electric organ of the torpedo fish. As depicted in Figure 5, This receptor consists of five subunits that form a pentagon, creating a central channel across the postsynaptic neuron’s membrane. When the neurotransmitter binds to the receptor, this channel opens, allowing calcium ions to pass through and generate a small electrical current. Thus, the electrical signal from the presynaptic neuron is converted into a chemical signal and then back into an electrical signal in the postsynaptic neuron. Voilà—this is how a neural message is transmitted across the synapse. Amazing, isn’t it?
Synaptopathies
What are Synaptopathies? Synaptopathies are conditions where the primary symptoms arise from disruptions in synapse formation and function [5]. These include certain forms of autism, severe learning disabilities, and schizophrenia. Advances in human genome sequencing have revealed many neurodevelopmental and psychiatric conditions linked to specific gene mutations. In some cases, single-gene mutations leading to gene silencing or deletion are identified as the causes. These findings make them particularly suitable for research, especially in animal models due to their relative simplicity. (Cf. previous blog entitled as: Brain Plasticity – I: Synapses – The Mushrooms of Learning).
Synaptopathies and Neurodevelopmental/Neuropsychiatric Disorders
Here are some established facts linking synaptopathies to neurodevelopmental and neuropsychiatric conditions:
- Synaptopathies often result from mutations in genes encoding synaptic proteins, which disrupt normal synaptic function. These mutations can lead to various neurodevelopmental and neuropsychiatric disorders.
- Autism spectrum disorder (ASD) is frequently associated with synaptopathies. Many individuals with ASD exhibit synaptic abnormalities, contributing to the core symptoms of social dysfunction, repetitive behaviors, and sensory sensitivities.
- There is a notable association between synaptopathies and epilepsy. Epilepsy is common in individuals with neurodevelopmental disorders like ASD, and synaptic dysfunction is believed to play a role in the development of seizures.
- Synaptic abnormalities have also been implicated in schizophrenia and bipolar disorder. These conditions often involve disruptions in synaptic signaling, which can affect cognitive and emotional processing.
- Synaptic plasticity, the ability of synapses to strengthen or weaken over time, is crucial for learning and memory. Synaptopathies can impair this plasticity, leading to learning difficulties and cognitive deficits in neurodevelopmental and neuropsychiatric conditions.
- Understanding synaptopathies opens up potential therapeutic avenues. Targeting synaptic dysfunction with drugs or other interventions could offer new treatments for these disorders.
These facts highlight the critical role of synaptic function in neurodevelopmental and neuropsychiatric conditions, and the potential for developing targeted therapies to address these disorders.
Spinogenesis
What is spinogenesis? Spinogenesis refers to the development of dendritic spines, which are closely linked to synaptogenesis due to the correlation between the number of spines and synapses [6]. However, there are differences between the two processes. While dendritic spines form in minutes, the formation of mature synapses can take days or weeks. Additionally, spinogenesis can occur independently of axonal contact, as seen in certain cellular contexts where dendritic spines develop without synaptic contact [7]. (Cf. previous blog entitled as: Brain Plasticity – I: Synapses – The Mushrooms of Learning).
The significance of spinogenesis is highlighted by the association between neurodevelopmental disorders and changes in dendritic spine number and morphology. Disorders such as schizophrenia, autism, Fragile X syndrome, Rett syndrome, and Down syndrome are characterized by reduced spine density and altered spine morphology. For instance, individuals with Fragile X syndrome exhibit long, immature spines, while those with Rett syndrome show reduced spine density and fewer mushroom-shaped spines. In Down syndrome, spine density decreases after 4 months of age, with remaining spines having large heads, indicating excessive pruning of synaptic connections during early postnatal development. These findings emphasize the importance of spinogenesis in brain development and its implications for various neurodevelopmental disorders [8]. (Cf. previous blogs entitled as: The Neurotypical Brain versus the Autistic Brain – Neurotypical Brain Development and Function; Defining Autism Spectrum Disorders: Mechanisms of Developmental Regression in Autism – II).
Spinogenesis and Neurodevelopmental/Neuropsychiatric Disorders
Here are some established facts about spinogenesis and its link to neurodevelopmental and neuropsychiatric conditions:
- Spinogenesis refers to the formation of dendritic spines, which are small protrusions on the dendrites of neurons where synapses form. These spines play a crucial role in synaptic connectivity and plasticity.
- Abnormal spinogenesis is often observed in neurodevelopmental disorders such as Autism Spectrum Disorder (ASD) and Fragile X Syndrome. These conditions are characterized by altered spine density and morphology, which can affect synaptic function and lead to cognitive and behavioral impairments.
- Spinogenesis is also implicated in neuropsychiatric disorders like schizophrenia and bipolar disorder. In these conditions, abnormalities in dendritic spine formation and maintenance can disrupt neural circuits, contributing to symptoms such as cognitive deficits and mood disturbances.
- Proper spinogenesis is essential for synaptic plasticity, the ability of synapses to strengthen or weaken over time in response to activity. This plasticity is fundamental for learning and memory, and disruptions in spinogenesis can impair these processes.
- Research into spinogenesis offers potential therapeutic targets for treating neurodevelopmental and neuropsychiatric conditions. By promoting healthy spine formation and maintenance, it may be possible to improve synaptic function and alleviate symptoms associated with these disorders.
These facts underscore the importance of spinogenesis in brain development and its potential role in various neurological and psychiatric conditions.
Take Home Messages
Ramón y Cajal: The Architect of Neuronal Theory and Artistic Pioneer in Neuroscience
- Santiago Ramón y Cajal revolutionized neuroscience by establishing the neuronal theory, demonstrating that the brain is made up of distinct neurons rather than a continuous network.
- His meticulous studies and artistic drawings of neurons remain foundational, inspiring generations of neuroscientists.
Decoding the Connectome: Unraveling the Brain’s Intricate Network
- Mapping the brain’s connectivity matrix, or ‘connectome,’ is crucial for understanding brain function.
- Efforts like those at the Allen Institute aim to provide a comprehensive map of neuron types and their connections, enhancing our understanding of the brain’s intricate network.
Neurotransmitter Symphony: The Molecular Dance of Neuronal Communication
- Neurons communicate through chemical messengers called neurotransmitters, which cross synapses to transmit signals.
- This process involves the release, diffusion, and binding of neurotransmitters to receptor molecules, enabling the conversion of electrical signals to chemical signals and back.
Synaptogenesis: Neurodevelopmental/Neuropsychiatric Disorders
- Synaptopathies are disorders resulting from mutations in synaptic genes, affecting synapse structure and function.
- These disorders are linked to neurodevelopmental and neuropsychiatric conditions like autism, schizophrenia, and severe learning disabilities.
Spinogenesis: Neurodevelopmental/Neuropsychiatric Disorders
- Spinogenesis, the development of dendritic spines, is crucial for synaptic connectivity and plasticity.
- Abnormalities in spinogenesis are linked to neurodevelopmental disorders like autism and neuropsychiatric conditions like schizophrenia, impacting cognitive and behavioral functions.
Summary and Conclusions
In this blog, our discussions briefly highlighted the intricate connections between neuronal theory, synaptic health, and brain function. Santiago Ramón y Cajal’s work laid the foundation for understanding the brain as a network of distinct neurons. Efforts to map the brain’s connectome, like those at the Allen Institute, are crucial for deciphering neural interactions. Neurons communicate through a complex process of neurotransmitter exchange, essential for brain activities. Synaptic dysfunction, or synaptopathies, is linked to neurodevelopmental and neuropsychiatric conditions, emphasizing the need for research in this area. Additionally, the development and maintenance of dendritic spines (spinogenesis) are vital for synaptic connectivity and plasticity, with abnormalities impacting cognitive and behavioral functions in various disorders. Understanding synaptic health is key to grasping brain plasticity, the brain’s remarkable ability to adapt and rewire itself in response to experiences, learning, and even injury. This underscores the importance of ongoing research to unlock the brain’s full potential!
For information on autism monitoring, screening and testing please read our blog.
References
- Katz-Sidlow RJ. The formulation of the neuron doctrine: the Island of Cajal. Arch Neurol. 1998 Feb;55(2):237-40. doi: 10.1001/archneur.55.2.237. PMID: 9482368.
https://pubmed.ncbi.nlm.nih.gov/9482368/ - Garcia-Lopez P, Garcia-Marin V, Freire M. The histological slides and drawings of cajal. Front Neuroanat. 2010 Mar 10;4:9. doi: 10.3389/neuro.05.009.2010. PMID: 20339483; PMCID: PMC2845060.
https://pubmed.ncbi.nlm.nih.gov/20339483/ - The MICrONS Consortium, et al. Functional connectomics spanning multiple areas of mouse visual cortex. doi:
https://doi.org/10.1101/2021.07.28.454025;
https://www.microns-explorer.org/cortical-mm3;
https://www.biorxiv.org/content/10.1101/2021.07.28.454025v3.full.pdf - Changeux JP. Discovery of the First Neurotransmitter Receptor: The Acetylcholine Nicotinic Receptor. Biomolecules. 2020 Apr 3;10(4):547. doi: 10.3390/biom10040547. PMID: 32260196; PMCID: PMC7226243.
https://pubmed.ncbi.nlm.nih.gov/32260196/ - Wang H, Balice-Gordon R. Editorial: Synaptic Diseases: From Biology to Potential Therapy. Front Synaptic Neurosci. 2022 Mar 9;14:846099. doi: 10.3389/fnsyn.2022.846099. PMID: 35480634; PMCID: PMC9037745.
https://pubmed.ncbi.nlm.nih.gov/35480634/ - Yuste R, Bonhoeffer T. Genesis of dendritic spines: insights from ultrastructural and imaging studies. Nat Rev Neurosci. 2004 Jan;5(1):24-34. doi: 10.1038/nrn1300. PMID: 14708001.
https://pubmed.ncbi.nlm.nih.gov/14708001/
- García-López P, García-Marín V, Freire M. Dendritic spines and development: towards a unifying model of spinogenesis–a present day review of Cajal’s histological slides and drawings. Neural Plast. 2010;2010:769207. doi: 10.1155/2010/769207. Epub 2011 Mar 13. PMID: 21584262; PMCID: PMC3091278.
https://pubmed.ncbi.nlm.nih.gov/21584262/
- Sala C, Segal M. Dendritic spines: the locus of structural and functional plasticity. Physiol Rev. 2014 Jan;94(1):141-88. doi: 10.1152/physrev.00012.2013. PMID: 24382885.
https://pubmed.ncbi.nlm.nih.gov/24382885/