Table of Contents
- Introduction
- Bacterial Transport: The Gatekeepers of Life
- The Power of Proton Gradients
- The Chemiosmotic Revolution: From Skepticism to Triumph
- The Nanomachine That Powers Life
- The Hidden Power of Respiration
- Bacteria: Nature’s Proton-Powered Machines
- The Origin of Life: Born from Proton Gradients?
- Take-Home Messages
- Summary and Conclusions
- Did You Know About Folate Receptor Autoantibodies (FRAAs) and Brain Development?
- References
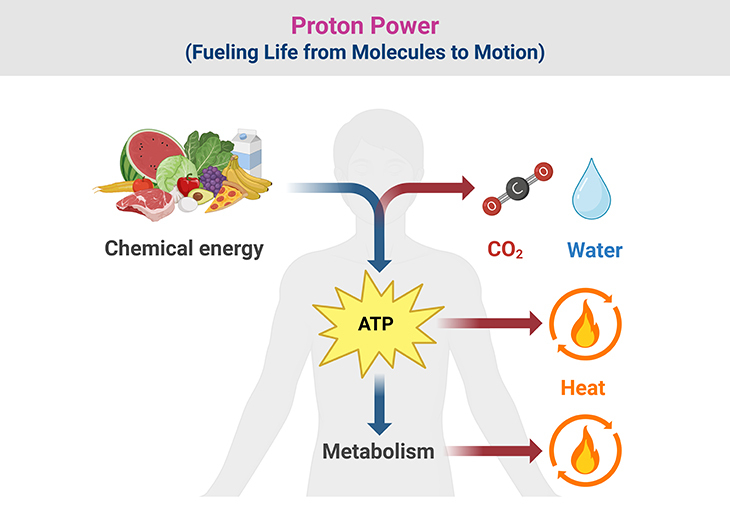
Figure 1. Proton Power: Fueling Life from Molecules to Motion. (1) From the moment food enters the body, it embarks on an intricate biochemical journey—a transformation of chemical energy into usable cellular power. Macronutrients like carbohydrates, fats, and proteins are broken down through metabolic pathways, where their stored energy is gradually released in a series of controlled redox reactions. These reactions fuel the electron transport chain, shuttling electrons through respiratory complexes and driving the pumping of protons across the mitochondrial membrane. This movement establishes a proton gradient, a charged reservoir of potential energy known as the proton-motive force. Like water held back by a dam, this force builds up, waiting to be harnessed for ATP production. (2) As protons flow back through ATP synthase, their energy is converted into adenosine triphosphate (ATP)—the universal fuel that powers everything from muscle contractions to enzyme activity. But this energy transfer is not perfectly efficient; some is lost as heat, a natural consequence that contributes to thermoregulation and metabolic balance. Meanwhile, the final products of respiration—water and carbon dioxide—mark the completion of this energy cycle, with CO₂ expelled through breathing and H₂O playing critical roles in cellular function. (3) This seamless interplay of food, metabolism, proton gradients, ATP synthesis, and energy dissipation underscores the remarkable efficiency of life’s energy systems. At its heart lies proton power, the molecular engine that sustains cellular function, ensuring that organisms can convert the nutrients they consume into the energy they need to thrive.
Introduction
The Energy That Powers Life
From the smallest bacteria to the largest mammals, every living cell depends on adenosine triphosphate (ATP)—the universal energy currency of life. ATP fuels the biochemical reactions that sustain organisms, from muscle contraction and metabolism to enzyme activation and cell division (see Figure 1). While its role in energy transfer is well understood today, the mechanism behind its synthesis remained one of the greatest puzzles in bioenergetics for much of the 20th century.
For decades, scientists sought a high-energy intermediate—an elusive molecule linking electron transport to ATP production. The prevailing assumption was that respiration followed a straightforward chemical coupling process, much like fermentation. But the search led nowhere. Instead, it took the unconventional thinking of Peter Mitchell to revolutionize the field. In 1961, Mitchell proposed the chemiosmotic hypothesis, suggesting that ATP synthesis was powered not by a direct chemical reaction but by proton gradients across membranes—a concept as radical then as it is fundamental today.
The chemiosmotic hypothesis not only solved longstanding paradoxes in bioenergetics but also unified respiration, photosynthesis, and bacterial metabolism under a single principle. This realization—that all energy conversion in living cells relies on proton-motive force—was met with skepticism, triggering heated scientific debates known as the “Ox Phos Wars.” It would take decades of experimental evidence, from isolated membrane studies to ATP production in artificial vesicles, to finally prove Mitchell right.
The journey to understanding ATP synthesis is one of controversy, breakthrough, and paradigm-shifting discovery. This article will explore the molecular mechanisms that drive life’s energy currency, tracing the experimental milestones that validated Mitchell’s ideas. From proton pumps and bacterial metabolism to ATP synthase—the nanomachine that powers life—this is the story of how proton gradients became biology’s hidden engine, shaping the way cells generate and utilize energy.
Bacterial Transport: The Gatekeepers of Life
Peter Mitchell’s fascination with bacteria revolved around a fundamental puzzle: how do cells import and export molecules, often against steep concentration gradients? Unlike passive diffusion, which allows molecules to flow freely, many essential nutrients and ions require active transport, a process demanding energy. Mitchell understood that the cell membrane was far more than an inert barrier—it was a dynamic, semi-permeable structure carefully regulating molecular exchange. He likened this process to an enzyme’s specificity: just as an enzyme recognizes a particular substrate, membrane proteins selectively transport molecules, ensuring cells receive what they need while expelling waste.
Bioenergetics and the Power of Gradients: During his time at Cambridge and later in Edinburgh, Mitchell expanded his thinking beyond bacterial transport to the deeper question of how cells harness energy. He realized that concentration gradients could be more than just barriers—they could store energy, much like air trapped inside a balloon. If a pump establishes a gradient, the resulting force could potentially drive molecular movement. This idea, inspired by everyday physics—such as the propulsion of a balloon by escaping air—pushed Mitchell toward the field of bioenergetics, linking bacterial physiology with biochemical energy conversion [1-3].
Chemiosmotic Coupling – A Revolutionary Hypothesis: In 1961, Mitchell put forth a radical new hypothesis that would fundamentally challenge biochemical dogma. He proposed that respiration did not rely on elusive chemical intermediates, as previously thought, but instead operated through chemiosmotic coupling. While most scientists associated osmosis with water movement, Mitchell took the term back to its Greek origins—meaning “push.” He argued that respiration’s true function was to push protons across a membrane, creating a reservoir of trapped energy. The membrane itself acted as a dam, holding back protons until their controlled release drove ATP synthesis (See Figure 2, 3 and 4).
The Proton-Motive Force – Nature’s Hidden Battery: Mitchell’s model explained how electron transport powers ATP production. Electrons, stripped from hydrogen atoms, travel down a chain of redox reactions, releasing energy. Instead of forming a mysterious high-energy intermediate, Mitchell proposed that this energy was used to pump protons across the mitochondrial membrane, creating a proton gradient. Because protons carry a positive charge, the buildup of protons establishes both an electrical potential and a pH difference across the membrane. Mitchell termed this stored energy “proton-motive force”—a force capable of driving ATP synthesis, much like a hydroelectric dam releasing water to turn turbines (see Figure 2, 3, and 4) [4-7].
Figure 2. Electron Transport and Proton Power: The Engine of ATP Synthesis. (1) The electron transport chain (ETC) is the molecular power grid of respiration, linking electron transfer to proton pumping and ATP synthesis. Electrons (e⁻) enter via Complex I or Complex II and are shuttled to Complex III by ubiquinone (Coenzyme Q, CoQ), a mobile carrier often marketed for health benefits despite its uncertain efficacy. Electrons then move to Complex IV (cytochrome oxidase) via cytochrome c (Cyt c), where they combine with protons (H⁺) and oxygen (O₂) to form water (H₂O), completing the electron flow. (2) Critically, as electrons cascade down the chain, the energy released powers proton translocation across the membrane, generating a proton gradient—an electrochemical reservoir of potential energy. This results in a measurable difference in proton concentration, which manifests as both a pH gradient (acidity difference) and an electrical potential difference, since protons carry a single positive charge. The proton gradient acts as an energy reservoir, akin to water held back behind a dam, ready to be unleashed for controlled energy conversion. (3) The flow of protons back through ATP synthase (Complex V) drives the proton-motive force, turning the molecular rotary motor and enabling ATP formation from ADP and phosphate (Pᵢ). This seamless coupling between electron transport, proton movement, and ATP synthesis underscores the fundamental role of proton power in bioenergetics, linking respiration, photosynthesis, and bacterial metabolism under a shared biochemical framework.
The Proton Electricity Controversy – The ‘Ox Phos’ Wars: Despite its elegance, Mitchell’s chemiosmotic theory was met with fierce resistance. Many researchers dismissed it outright, refusing to abandon the idea of a high-energy chemical intermediate. Others regarded Mitchell’s work as derivative or even delusional. The heated debates, spanning nearly two decades, became known as the ‘ox phos’ wars—a scientific battle over how cells truly generate ATP. Eventually, as experimental evidence mounted, Mitchell’s theory triumphed, reshaping bioenergetics and earning him the Nobel Prize in 1978.
A Visionary Who Redefined Bioenergetics: Today, Mitchell’s chemiosmotic hypothesis stands as one of the most transformative ideas in molecular biology. His vision turned ATP synthesis from a biochemical enigma into an elegant, gradient-driven energy conversion system. By redefining how cells harness energy, Mitchell’s work forever changed our understanding of metabolism, bioenergetics, and the fundamental processes that power life itself.
The Power of Proton Gradients
Peter Mitchell’s chemiosmotic hypothesis elegantly resolved longstanding mysteries in bioenergetics, particularly the necessity of an intact membrane. Without a barrier, protons would leak back uncontrollably, dissipating the proton-motive force as heat—much like water seeping through a damaged dam. This explained why respiration required a fully functional membrane, and why disruptions led to a collapse in ATP production.
Uncoupling – The Broken Link Between Energy and Work: Mitchell’s hypothesis also shed light on the enigmatic uncoupling agents, which severed the link between glucose oxidation and ATP synthesis—similar to a bicycle losing its chain. Previously, these agents appeared unrelated, but Mitchell identified a commonality: they were weak acids capable of dissolving into the membrane’s lipids. These acids shuttled protons across the membrane by alternating between protonated and deprotonated states, effectively short-circuiting the proton gradient. This mechanistic insight clarified why some compounds uncoupled respiration while others failed—their ability to remain lipid-soluble, regardless of proton binding, determined their effectiveness.
The Proton-Motive Force – Energy Without an Intermediate: One of Mitchell’s most groundbreaking insights was his rejection of a high-energy intermediate, often referred to as the elusive “squiggle.” Instead, he demonstrated that protons pumped across the membrane in one location could drive ATP synthesis elsewhere, much like water behind a dam exerting equal pressure across the entire structure. This direct energy conversion via proton-motive force explained why ATP generation did not require a fixed electron-to-ATP ratio—proton leaks and alternative proton-consuming processes contributed to variations in ATP yield.
Experimental Proof – The Voltage Across Life’s Power Source: Perhaps most importantly, Mitchell’s theory was testable. Over the next decade, alongside Jennifer Moyle at Glynn House, Mitchell provided concrete evidence that mitochondria generate both a pH gradient and an electrical charge (~150 millivolts) across the inner membrane. While this voltage seems modest compared to household batteries, in molecular terms, it equates to 30 million volts per meter—comparable to a lightning bolt. Further experiments confirmed:
- A spike in oxygen levels correlated with increased proton pumping.
- Uncoupling agents actively shuttled protons across membranes, proving the mechanism.
- The proton-motive force directly powered ATP synthase, fulfilling Mitchell’s predictions.
- Proton pumping depended on the movement of electrons down the respiratory chain and stalled when key substrates such as hydrogen atoms, oxygen, ADP, or phosphate ran short.
The Chemiosmotic Revolution: From Skepticism to Triumph
Initially dismissed and even ridiculed, Mitchell’s hypothesis ignited fierce debates, famously known as the ‘Ox Phos’ Wars’ (from oxidative phosphorylation). Many researchers clung to the idea of a traditional high-energy intermediate, resisting the concept of proton-driven energy transfer. However, as experimental validation piled up, the scientific community gradually embraced chemiosmotic coupling, solidifying it as one of the most profound shifts in bioenergetics.
A Paradigm Shift in Cellular Energy: Mitchell’s work did more than solve biochemical puzzles—it redefined energy conversion in living systems. His vision of proton gradients as cellular batteries not only unraveled the mechanism behind respiration but also paved the way for deeper explorations into photosynthesis, bacterial metabolism, and even emerging biomedical applications. Today, his chemiosmotic theory stands as a cornerstone of biological energy dynamics, a testament to the power of a bold, unconventional idea.
Convincing the Skeptics – Experimental Breakthroughs: As the chemiosmotic hypothesis gained traction, Peter Mitchell and Jennifer Moyle were no longer alone in their experimental efforts. Efraim Racker played a pivotal role in validating the theory, demonstrating that isolated respiratory complexes embedded in artificial lipid vesicles could still generate a proton gradient. This reinforced the idea that membranes were essential for energy conversion, even outside intact cells.
Yet, the experiment that truly shook the field, particularly among botanists, came from André Jagendorf and Earnest Uribe at Cornell University in 1966. Working with chloroplast membranes, they exposed them to an acidic solution (pH 4) and allowed equilibrium to set across the membrane. Then, they injected an alkaline solution (pH 8), creating a sharp proton gradient. The result? ATP was synthesized without light or any other energy source—powered solely by the proton difference.
This striking finding unified respiration and photosynthesis, revealing that both relied on the same proton-motive force to drive ATP production, despite their vastly different origins. It was a major vindication of Mitchell’s theory, and in 1978, he was awarded the Nobel Prize in Chemistry for transforming bioenergetics.
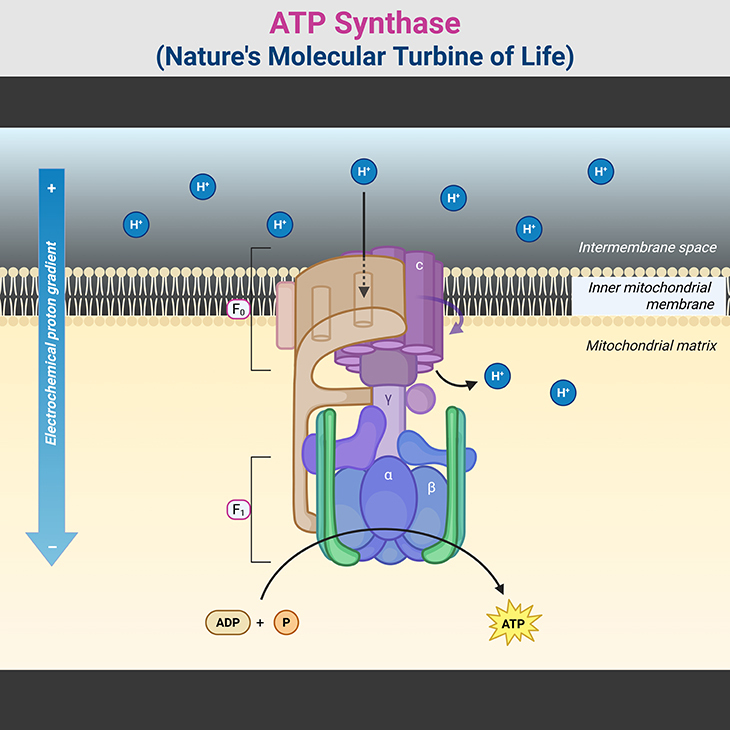
Figure 3. ATP Synthase: Nature’s Molecular Turbine of Life. (1) ATP synthase, a molecular rotary engine, is a masterpiece of biological engineering that converts proton-motive force into ATP, the universal energy currency of life. This remarkable nanomachine is composed of two distinct domains: F₀, the membrane-embedded proton motor, and F₁, the enzymatic core responsible for ATP synthesis. These domains work in perfect synchrony, ensuring efficient energy conversion across all domains of life. (2) The F₀ domain acts as a proton-driven turbine, embedded within the mitochondrial or bacterial membrane. A ring of c-subunits forms the rotary proton channel, allowing H⁺ ions to pass through, powering rotational motion. The a-subunit channels protons to specific binding sites on the c-ring, ensuring controlled movement, while the b-subunit and δ-subunit provide essential structural support, anchoring the catalytic core in place. (3) Connected to F₀ is the F₁ domain, where ATP synthesis occurs. At its heart lies a hexameric ring of alternating α and β-subunits, forming three catalytic sites for ATP generation. The γ-subunit acts as a central axle, transmitting rotational motion from F₀ to F₁, inducing conformational changes in the β-subunits to drive ATP formation. Supporting subunits like ε and δ regulate rotation, preventing uncontrolled ATP hydrolysis. (4) As protons flow through F₀, they rotate the c-ring, setting the entire nanomachine into motion. This rotation induces structural changes in the β-subunits, cycling them through three distinct states—open, loose, and tight—to convert ADP and inorganic phosphate (Pᵢ) into ATP. Each full 360° rotation of the γ-subunit produces three ATP molecules, sustaining cellular processes with remarkable efficiency. (5) The molecular precision of ATP synthase is astounding—it operates at near-perfect energy conversion, rivaling engineered turbines. A single human mitochondrion produces thousands of ATP molecules per second, and the body generates approximately its own weight in ATP daily to fuel essential functions. Its presence across bacteria, archaea, and eukaryotes underscores its evolutionary importance, dating back to the earliest bioenergetic systems. (6) Even more astonishing is ATP synthase’s ability to reverse function when necessary. Under metabolic stress, when ATP levels drop, this nanomachine switches direction, consuming ATP to restore the proton gradient—a survival mechanism vital for bacterial adaptation. (7) This nano-motor of life exemplifies nature’s engineering brilliance, seamlessly translating proton power into life-sustaining energy. Its elegance and efficiency demonstrate why ATP synthase remains one of biology’s most fundamental and awe-inspiring molecular machines.
The Nanomachine That Powers Life
Since Mitchell’s Nobel win, researchers have meticulously unraveled the finer details of electron transport, proton pumping, and ATP synthesis. The most stunning breakthrough came when John Walker determined the atomic structure of ATP synthase, earning him the Nobel Prize in 1997, alongside Paul Boyer, who had theorized its rotary mechanism decades earlier (see Figure 3 and 4) [4-7, 10].
ATP synthase is nature’s ultimate nanomachine, constructed from tiny moving protein parts. It functions like a microscopic rotary motor, consisting of:
- A drive shaft, embedded straight through the membrane.
- A rotating head, resembling a mushroom cap under an electron microscope.
The pressure of protons trapped outside the membrane forces them through the drive shaft, causing the rotating head to crank around by 120° per three protons. Each full turn completes three rotations and results in ATP formation.
How ATP is Built – The Three-Step Cycle: The ATPase operates with three distinct binding sites, each responsible for a different stage of ATP synthesis:
- Binding ADP at the first site.
- Attaching phosphate (Pᵢ) to ADP in the second site, forming ATP.
- Releasing ATP at the third site, completing the process.
In humans, a full turn of the ATP synthase head requires 10 protons and produces 3 molecules of ATP. Interestingly, different species require varying numbers of protons per rotation, adding complexity to its universal role in bioenergetics.
ATPase in Reverse – A Hidden Secret of Life: Perhaps the most fascinating aspect of ATP synthase is its reversibility. Under certain conditions, it switches directions—splitting ATP instead of forming it and using the released energy to pump protons back across the membrane.
This backward function, first discovered before its role in ATP synthesis, is the reason behind the enzyme’s original name—ATPase, rather than ATP synthase. This curious ability holds a deep secret about life’s fundamental energy balance—one that we will explore further soon.
The Machinery That Runs the Cell: From Mitchell’s radical hypothesis to Walker’s atomic-level insights, the journey to understand ATP synthesis has been one of controversy, breakthrough, and scientific triumph. ATP synthase stands as a marvel of molecular engineering, a nanomachine with precision mechanics, quietly turning within every living cell to generate the energy that sustains life (see Figure 3 and 4).
Figure 4. ATP Synthesis: ATP Synthase Rotation. {Image credits – Animations from Wikipedia, The Free Encyclopedia (left panel); and Zhou et al. 2015 (right panel)} [File:ATP synthesis – ATP synthase rotation.ogv – Wikipedia; Structure and conformational states of the bovine mitochondrial ATP synthase by cryo-EM | eLife] [10]
The Hidden Power of Respiration
At its core, respiration is an energy-harnessing process driven by proton pumps. As electrons flow through the respiratory chain, redox reactions release energy, which is used to pump protons across a membrane. This creates a proton gradient, corresponding to an electrical charge of roughly 150 mV, known as the proton-motive force. This stored energy drives ATP synthase, the molecular motor responsible for producing ATP—the universal energy currency of life.
Proton Power – A Common Thread in Biology: Surprisingly, photosynthesis operates on the same fundamental principle—except instead of extracting energy from chemical oxidation, it harnesses sunlight to pump protons across the chloroplast membrane, creating a proton gradient that fuels ATP production. Bacteria, too, exploit this mechanism, generating proton-motive force across their outer cell membrane.
For those unfamiliar with microbiology, bacterial energy generation may seem chaotic—they extract energy from methane, sulfur, even concrete! But beneath this astonishing biochemical versatility lies a unified principle: electrons flow down a redox chain to a terminal electron acceptor (which may be CO₂, NO₃⁻, SO₄²⁻, Fe³⁺, or oxygen), and the energy released pumps protons across a membrane, establishing a proton-motive force.
The Universal Signature of Life: This deep unity across biological energy systems is remarkable—not just for its universality, but for its unconventional nature. As Leslie Orgel famously remarked, “Few would have laid money on cells generating energy with proton pumps.” And yet, across respiration, photosynthesis, and bacterial metabolism, proton pumping remains a defining feature of life—as fundamental as DNA itself [8-9].
Proton Gradients – The Force Field of Cellular Life: Peter Mitchell recognized that the proton-motive force was not limited to ATP production—it extended into active transport systems, enveloping bacteria in an invisible source of power. Many membrane transporters do not use ATP directly—instead, they extract energy from the proton gradient to move molecules against their concentration gradients.
For example:
- Lactose import: A membrane pump binds one lactose molecule and one proton—so instead of consuming ATP, the proton gradient itself fuels lactose transport into the cell.
- Sodium export: Removing one sodium ion costs one proton, again dissipating the gradient without using ATP.
This mechanism allows bacteria to economically regulate nutrient intake, conserving ATP for other crucial processes.
Proton Gradients as a Source of Heat: Sometimes, cells deliberately dissipate proton gradients—not for ATP synthesis, but to generate heat. In such cases, respiration becomes uncoupled—electron flow and proton pumping proceed without ATP production, as protons pass back through membrane pores rather than ATP synthase.
This serves a dual purpose:
- Heat production—which is biologically useful, such as in thermoregulation.
- Preventing electron congestion—reducing the risk of free radical formation.
Just as a hydroelectric dam prevents overflow by redirecting water through spillways, mitochondrial proton leak pathways help prevent the dangerous accumulation of electrons, which could otherwise react with oxygen and produce harmful free radicals.
A Profound and Elegant System: The more we dissect bioenergetics, the clearer it becomes: the proton-motive force is not just a mechanism—it is a fundamental signature of life. From ATP generation to nutrient transport, heat dissipation, and electron regulation, proton gradients sustain cellular function in ways previously unimaginable.
Through Mitchell’s visionary work, what once seemed an overly complicated, counterintuitive system emerged as one of life’s most elegant and efficient strategies for energy conversion.
Bacteria: Nature’s Proton-Powered Machines
While ATP is often hailed as the universal energy currency, bacteria rely heavily on proton power for survival. Franklin Harold and colleagues, working in the 1970s, revealed that bacterial locomotion is directly driven by the proton-motive force. Many bacteria propel themselves using corkscrew-like flagella, rotating at astonishing speeds—hundreds of cell lengths per second.
The engine behind this movement is a rotary motor protein, remarkably similar to ATP synthase, except instead of synthesizing ATP, it harnesses proton flow to power flagellar rotation. This insight expanded the role of proton gradients beyond ATP synthesis, demonstrating their importance in bacterial mobility and environmental adaptation.
The Proton Gradient – Life’s Hidden Battery: Bacteria are, in many ways, proton-driven organisms. Their homeostasis and locomotion depend on proton gradients rather than ATP alone. This explains why the respiratory chain pumps more protons than ATP synthase requires—because protons fuel multiple cellular functions beyond energy generation. This also accounts for the difficulty in quantifying ATP yield per electron, as various biological processes tap into the proton gradient, siphoning off some of its energy.
Why ATP Synthase Can Run in Reverse: A surprising feature of ATP synthase is its ability to reverse direction, breaking down ATP to pump protons back across the membrane. At first glance, this seems counterproductive—it rapidly depletes ATP reserves. However, this reversal serves a critical survival function, reinforcing the idea that maintaining the proton gradient is more vital than ATP storage itself.
When respiration falters, bacteria switch to fermentation, generating ATP through glycolysis. However, instead of using this ATP for reproduction or DNA replication, ATP synthase immediately reverses course, using freshly made ATP to recharge the proton gradient—an emergency energy reset, akin to a starship restoring its force field before battle.
Proton Pumping – A Primitive and Universal Mechanism: The central role of proton pumping hints at its deep evolutionary roots. More than a molecular quirk, it is a fundamental property of life, present across all three domains—bacteria, archaea, and eukarya. It drives respiration, photosynthesis, active transport, and movement, underscoring its unifying role in biology.
The Origin of Life: Born from Proton Gradients?
Given the critical importance of proton gradients, some researchers propose that life’s origins were intimately tied to natural proton fluxes. Hydrothermal vents, for example, create spontaneous proton gradients, potentially offering the first energy source for primitive biochemical reactions. If so, proton pumping may not only define life today—but may have played a central role in its very emergence.
Proton Power as Life’s Core Principle: From flagellar propulsion to fermentation-driven survival strategies, the proton-motive force is far more than a biochemical footnote—it is a signature of life itself. While ATP fuels cellular reactions, proton gradients power essential processes, reinforcing the notion that proton flux may have shaped the earliest cellular systems.
Take-Home Message
- ATP is life’s universal energy currency, powering essential biological functions.
- Proton-motive force is the hidden engine of bioenergetics, driving ATP synthesis, active transport, locomotion, and heat generation.
- Peter Mitchell’s chemiosmotic hypothesis revolutionized bioenergetics, proving that proton gradients—not elusive high-energy intermediates—power ATP production.
- Proton gradients unify respiration, photosynthesis, and bacterial metabolism, revealing a common principle across diverse energy systems.
- Beyond ATP synthesis, proton gradients fuel bacterial locomotion, enabling flagellar propulsion and environmental adaptation.
- Bacterial homeostasis relies on proton gradients, allowing nutrient import and waste removal without ATP expenditure.
- Proton dissipation regulates cellular energy flow, maintaining electron transport chain stability and reducing free radical damage.
- ATP synthase can run in reverse, depleting ATP to restore the proton gradient—a survival mechanism during metabolic stress.
- Proton gradients may have played a pivotal role in life’s origins, potentially serving as the first energy source for primordial biochemical reactions.
(Cf. previous blogs entitled as: “Cellular Respiration: The Hidden Engine Driving Life’s Energy.” “ATP: The Molecular Currency That Keeps Life Running.”)
Summary and Conclusions
The chemiosmotic hypothesis proposed by Peter Mitchell fundamentally reshaped our understanding of bioenergetics. His pioneering idea—that ATP synthesis is driven by proton gradients rather than high-energy intermediates—provided a unifying principle for respiration, photosynthesis, and bacterial metabolism. Overcoming deep skepticism, the theory was ultimately validated through key experiments, such as Jagendorf and Uribe’s chloroplast studies, which demonstrated ATP production driven solely by proton gradients. John Walker’s structural determination of ATP synthase further cemented the model, revealing the enzyme’s rotary mechanism that harnesses proton flow to generate ATP.
Beyond ATP synthesis, proton-motive force plays a broader physiological role, fueling bacterial locomotion, membrane transport, and even heat production. The discovery that bacteria prioritize maintaining proton gradients over ATP reserves underscores their evolutionary significance—suggesting that proton-driven energy systems may have existed since life’s earliest origins. These gradients are not merely biochemical mechanisms but a fundamental energy strategy across all domains of life.
Since Mitchell’s era, advances in structural biology, single-molecule studies, and bioenergetics have further refined our understanding of electron transport, proton dynamics, and cellular metabolism. The realization that proton gradients could have played a role in the prebiotic conditions of early Earth has fueled hypotheses connecting hydrothermal vents and geochemical energy sources to the emergence of life.
Thus, chemiosmotic coupling is far more than a molecular mechanism—it is the cornerstone of cellular energy conversion, shaping life’s most fundamental processes. Today, its implications extend into biomedicine, synthetic biology, and energy-harnessing technologies, reinforcing the power of proton gradients as a biological force field essential for survival and adaptation.
For information on autism monitoring, screening and testing please read our blog.
References
- Deshpande OA, Mohiuddin SS. Biochemistry, Oxidative Phosphorylation. [Updated 2023 Jul 31]. In: StatPearls [Internet]. Treasure Island (FL): StatPearls Publishing; 2025 Jan-. Available from:
https://www.ncbi.nlm.nih.gov/sites/books/NBK553192/ - Silverstein TP. The Proton in Biochemistry: Impacts on Bioenergetics, Biophysical Chemistry, and Bioorganic Chemistry. Front Mol Biosci. 2021 Nov 26;8:764099. doi: 10.3389/fmolb.2021.764099. PMID: 34901158; PMCID: PMC8661011.
https://pubmed.ncbi.nlm.nih.gov/34901158/ - Nirody JA, Budin I, Rangamani P. ATP synthase: Evolution, energetics, and membrane interactions. J Gen Physiol. 2020 Nov 2;152(11):e201912475. doi: 10.1085/jgp.201912475. PMID: 32966553; PMCID: PMC7594442.
https://pubmed.ncbi.nlm.nih.gov/32966553/ - Courbon GM, Rubinstein JL. CryoEM Reveals the Complexity and Diversity of ATP Synthases. Front Microbiol. 2022 Jun 16;13:864006. doi: 10.3389/fmicb.2022.864006. PMID: 35783400; PMCID: PMC9244403.
https://pubmed.ncbi.nlm.nih.gov/35783400/ - Dimroth P, von Ballmoos C, Meier T, Kaim G. Electrical power fuels rotary ATP synthase. Structure. 2003 Dec;11(12):1469-73. doi: 10.1016/j.str.2003.11.011. PMID: 14656431.
https://pubmed.ncbi.nlm.nih.gov/14656431/
- Neupane P, Bhuju S, Thapa N, Bhattarai HK. ATP Synthase: Structure, Function and Inhibition. Biomol Concepts. 2019 Mar 7;10(1):1-10. doi: 10.1515/bmc-2019-0001. PMID: 30888962.
https://pubmed.ncbi.nlm.nih.gov/30888962/ - Vercellino I, Sazanov LA. The assembly, regulation and function of the mitochondrial respiratory chain. Nat Rev Mol Cell Biol. 2022 Feb;23(2):141-161. doi: 10.1038/s41580-021-00415-0. Epub 2021 Oct 7. PMID: 34621061.
https://pubmed.ncbi.nlm.nih.gov/34621061/
https://bookcafe.yuntsg.com/ueditor/jsp/upload/file/20211216/1639624341251039230.pdf - Lane N. Mitochondrial disease: powerhouse of disease. Nature. 2006 Mar 30;440(7084):600-2. doi: 10.1038/440600a. PMID: 16572142.
https://pubmed.ncbi.nlm.nih.gov/16572142/ - Lane N, Martin WF. The origin of membrane bioenergetics. Cell. 2012 Dec 21;151(7):1406-16. doi: 10.1016/j.cell.2012.11.050. PMID: 23260134.
https://pubmed.ncbi.nlm.nih.gov/23260134/ - Zhou A, Rohou A, Schep DG, Bason JV, Montgomery MG, Walker JE, Grigorieff N, Rubinstein JL. Structure and conformational states of the bovine mitochondrial ATP synthase by cryo-EM. Elife. 2015 Oct 6;4:e10180. doi: 10.7554/eLife.10180. PMID: 26439008; PMCID: PMC4718723.
https://pubmed.ncbi.nlm.nih.gov/26439008/
https://elifesciences.org/articles/10180