Table of Contents
- Introduction
- What Causes Regression?
- Inflammation and Immune Dysregulation
- Epilepsy and Regression
- Infantile Proteopathy and Tauopathy
- Central Role of Synaptic Health
- Clinical Considerations
- Take Home Messages
- Conclusions
- Did You Know About Folate Receptor Autoantibodies (FRAAs) and Brain Development?
- References
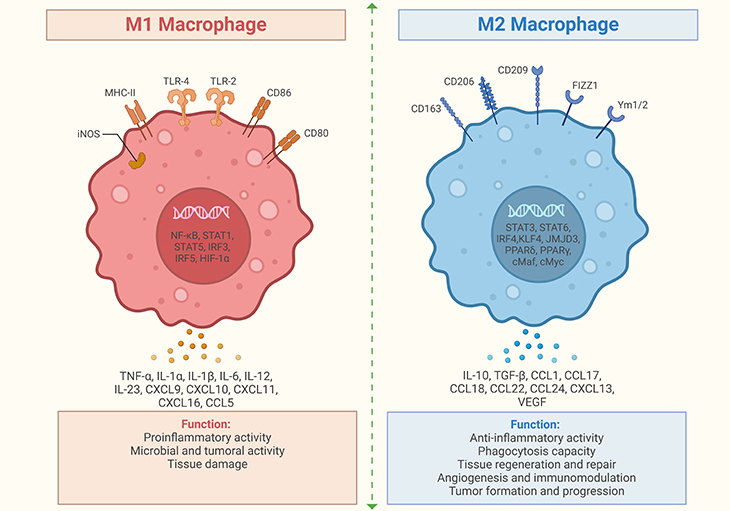
Figure 1. Role of M1 and M2 macrophages in neuroinflammation. (1) M1 macrophages: M1 macrophages produce pro-inflammatory cytokines such as tumor necrosis factor (TNF), interleukin-1 (IL-1), interleukin-6 (IL-6), and nitric oxide (NO). They are typically activated by microbial products like lipopolysaccharide (LPS) and pro-inflammatory cytokines such as interferon-gamma (IFN-γ). M1 macrophages are involved in the initial response to infection and tissue damage, promoting inflammation. (2) M2 macrophages: M2 macrophages produce anti-inflammatory cytokines such as interleukin-10 (IL-10), transforming growth factor-beta (TGF-β), and arginase-1 (Arg-1). They are typically activated by anti-inflammatory cytokines like interleukin-4 (IL-4) and interleukin-13 (IL-13). M2 macrophages are involved in tissue repair, wound healing, and the resolution of inflammation. (3) Role in neuroinflammation: In the brain, macrophages (including microglia) can adopt M1 or M2 phenotypes. M1 macrophages can exacerbate neuroinflammation, potentially leading to neuronal damage. M2 macrophages can aid in tissue repair and reduce inflammation, promoting recovery. These facts highlight the dual roles of M1 and M2 macrophages in inflammation and tissue repair, both in the brain and other tissues. [ https://pubmed.ncbi.nlm.nih.gov/31037072/] [1]
Introduction
Regressive autism, also known as autism with regression, is a subtype of Autism Spectrum Disorder (ASD). It is characterized by a significant loss of previously acquired skills and abilities, such as language, social skills, and other developmental milestones. Key characteristics include, for example:
- Loss of language: Children may stop using words they previously knew.
- Social withdrawal: Children may withdraw from social interactions.
- Repetitive behaviors: Engaging in repetitive actions or restricted interests.
- Sensory sensitivities: Increased sensitivity to sensory stimuli.
- Difficulties with transitions: Challenges in adapting to changes in routine or environment.
What Causes Regression?
The exact causes of regressive autism are still being studied, but several factors have been suggested as potential contributors, for instance:
- Genetic factors: Family history and genetic mutations may play a role.
- Environmental triggers: Exposure to certain environmental factors during critical periods of development.
- Neurodevelopmental factors: Abnormalities in brain development.
- Mitochondrial dysfunction: Issues with cellular energy production.
- Autoimmunity: The body’s immune response attacking its own cells.
- Infections: Certain infections during early childhood.
- Stress and mental health issues: High levels of stress or underlying mental health conditions.
It’s important to note that these factors may interact in complex ways, and more research is needed to fully understand the causes of regressive autism. (Cf. previous blog entitled as: “Defining Autism Spectrum Disorders: Mechanisms of Developmental Regression in Autism”).
Inflammation and Immune Dysregulation
I. Immune dysregulation
The relationship between autism and immune dysregulation has been investigated for decades, and the mechanisms of risk are potentially complex. Early research identified a high prevalence of autoimmune disorders in families of individuals with autism spectrum disorders (ASDs). This suggests a potential genetic or environmental link between immune dysregulation and autism.
Meanwhile, more recent studies have found that mothers, autistic children, and their siblings produce higher numbers of autoantibodies targeting brain components like the myelin sheath [2]. These autoantibodies can potentially cross the placental and fetal blood-brain barriers, affecting the developing brain.
Even though it is possible this a related yet incidental finding of familial immune dysfunction, now, we do know that maternal IgG antibodies are capable of passing through the placenta and fetal blood-brain barriers, suggesting that autoantibodies can access the embryonic brain and potentially increase autism risk. Primate studies have shown that prenatal exposure to autoantibodies from mothers of autistic children can lead to socially abnormal behavior in monkeys. This provides a model for understanding how maternal autoantibodies might affect brain development and behavior.
At the same time, immune factors known as cytokine offer another potential avenue for autism risk. For instance, cytokines, such as interleukin-6 (IL-6), play a role in immune responses and can cross the placental barrier. Maternal infection during pregnancy, which increases cytokine levels, has been linked to a higher risk of autism. IL-6, in particular, can alter neuron development (both during prenatal development as well as in the adult hippocampus in which neuron regeneration occurs lifelong) and stimulate immune cells that may trigger autoimmunity within the brain (see Figure 1; Figure 2) [3].
These points emphasize the intricate interplay between immune factors and neurodevelopment, suggesting that maternal autoimmunity and infections during pregnancy could contribute to autism risk.
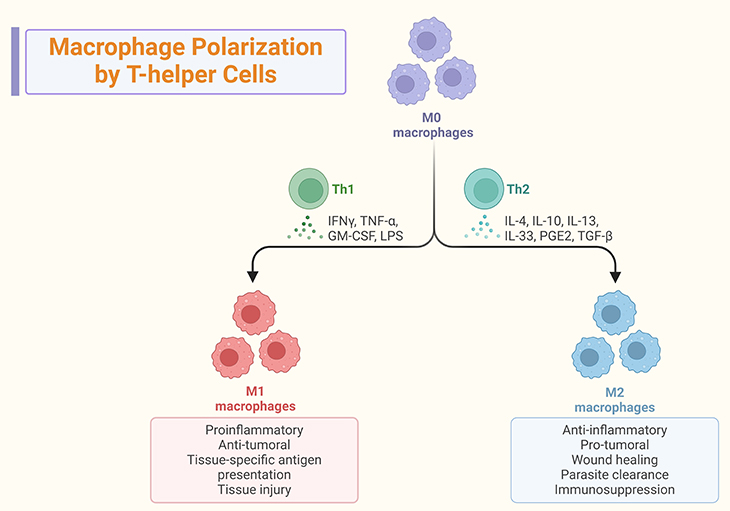
Figure 2. Macrophage Polarization by T-helper Cells. (1) Macrophage polarization: M1 Macrophages are pro-inflammatory macrophages that produce cytokines like IL-12, promoting a Th1 (T-helper 1) response. They are involved in the initial immune response to pathogens and tissue damage. M2 Macrophages are anti-inflammatory macrophages that produce cytokines like IL-4 and IL-13, promoting a Th2 (T-helper 2) response. They are involved in tissue repair, wound healing, and the resolution of inflammation. (2) Role in inflammation: Th1 response – M1 macrophages and Th1 cells work together to enhance the inflammatory response, which is crucial for fighting infections but can also lead to tissue damage if not regulated. Th2 response – M2 macrophages and Th2 cells work together to suppress inflammation and promote healing, helping to restore tissue homeostasis. (3) Interaction with T-helper Cells: Dynamic Interaction – the interaction between T-helper cells and macrophages is dynamic and influences the type of immune response. M1 macrophages promote Th1 responses, while M2 macrophages promote Th2 responses. Adaptive Immune Response – this interaction is crucial for the adaptive immune response, as it helps tailor the immune response to specific pathogens and conditions. These facts highlight the critical role of macrophage polarization in shaping the immune response and maintaining tissue homeostasis.
II. Inflammation
Inflammation, while a protective response, can cause significant neuronal damage if excessive or prolonged. This is well-documented in various neurodegenerative diseases, for example, adult forms of dementia [4].
Studies have shown that autistic children who regress are more likely to have experienced fever-related illnesses six months prior to regression. Additionally, families of children with regressive autism have higher rates of autoimmune disorders (33 percent vs. 12 percent), suggesting a heritable immune component.
A clear-cut case of immune-related regression can be discerned in the genetic condition known as Dravet syndrome (see Box-1). This genetic condition is a form of epileptic encephalopathy often accompanied by intellectual disability and autism. In addition, about 25 percent of Dravet syndrome patients have autism [5]. Children with Dravet syndrome typically develop normally until seizures begin, leading to regression in psychomotor skills.
Surprisingly, in Dravet syndrome, seizure onset is often preceded by illness or vaccination, indicating that immune challenges can trigger regression. However, the overall clinical outcomes do not significantly differ between those with and without such triggers, except for an earlier onset of seizures in the former group. Most individuals with Dravet syndrome have mutations in the SCN1A gene, which is a sodium channel gene. Clinical genotyping can provide early diagnosis and inform treatment decisions.
These points underscore the complex interplay between immune factors and neurodevelopment, highlighting the need for further research to better understand these connections and improve treatment strategies.
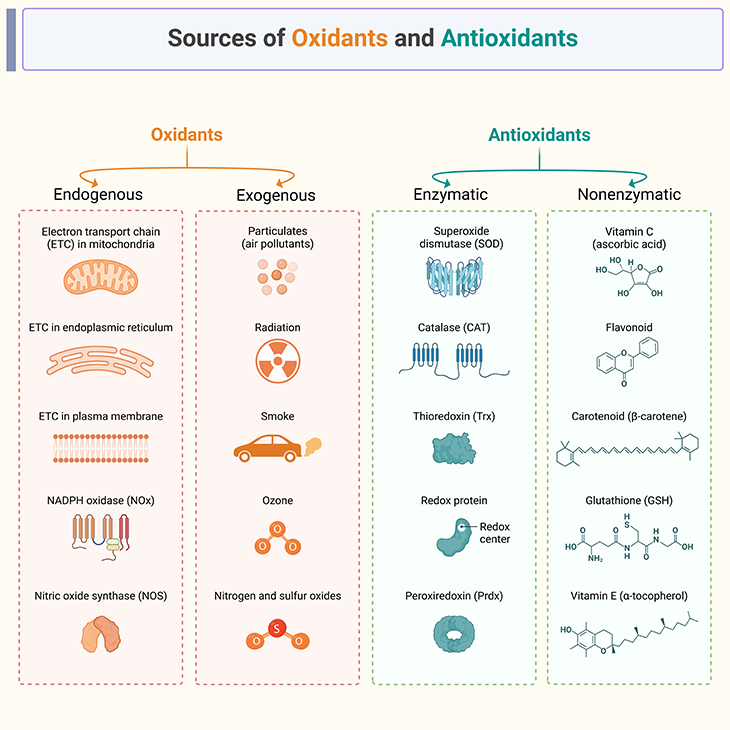
Figure 3. Sources of oxidants and antioxidants.
(A) Endogenous oxidants, also known as reactive oxygen species (ROS), are produced naturally within the body and play a role in various cellular processes. Here are some established sources of endogenous oxidants: (1) Mitochondrial Electron Transport Chain (Mito-ETC): During cellular respiration, electrons are transferred through the Mito-ETC, leading to the production of ROS such as superoxide anion (O2•−). (2) NADPH Oxidases (NOx): These enzymes generate ROS as part of the body’s immune response and other cellular signaling processes. (3) Xanthine Oxidase: Involved in purine metabolism, this enzyme produces hydrogen peroxide (H2O2) and superoxide anion. (4) Pro-inflammatory Cytokines: Cytokines like TNF-α and IL-1β can stimulate the production of ROS by immune cells. (5) Hypoxia: Low oxygen levels can lead to the production of ROS as cells attempt to adapt to the hypoxic environment. These endogenous oxidants are part of normal cellular functions but can cause damage if not regulated by the body’s antioxidant defenses.
(B) Exogenous oxidants are external sources of oxidative stress that can lead to cellular damage. Here are some established exogenous oxidants: (1) Pollution: Airborne pollutants such as particulate matter, ozone, and nitrogen dioxide can generate free radicals and oxidative stress. (2) Cigarette Smoke: Contains a variety of oxidizing chemicals, including free radicals and reactive oxygen species (ROS). (3) Radiation: Ultraviolet (UV) radiation from the sun and ionizing radiation from medical imaging or treatments can produce free radicals. (4) Industrial Chemicals: Certain chemicals used in manufacturing, such as benzene and formaldehyde, can act as oxidants. (5) Processed Foods: High levels of sugars and fats in processed foods can lead to increased oxidative stress. (6) Alcohol: Excessive alcohol consumption can generate ROS and deplete antioxidant defenses. (7) Infections: Pathogens and the body’s response to infections can produce ROS as part of the immune response. These exogenous oxidants contribute to oxidative stress, which can damage cells and tissues if not balanced by antioxidants.
(C) Endogenous antioxidants are produced naturally by the body and play a crucial role in protecting cells from oxidative stress. Here are some established endogenous antioxidants: (1) Glutathione (GSH): Often termed the body’s “master antioxidant,” it is vital for detoxification and immune system support. (2) Superoxide Dismutase (SOD): An enzyme that converts superoxide radicals into oxygen and hydrogen peroxide, reducing oxidative stress. (3) Catalase (CAT): An enzyme that breaks down hydrogen peroxide into water and oxygen, preventing cellular damage. (4) Coenzyme Q10 (CoQ10): Essential for energy production in mitochondria and acts as an antioxidant. (5) Alpha-Lipoic Acid (ALA): Functions in both water and fat-soluble environments and helps regenerate other antioxidants like vitamin C and E. (6) Uric Acid: A product of purine metabolism that has antioxidant properties and helps neutralize free radicals. (7) Melatonin: Known for regulating sleep, it also has antioxidant properties and protects cells from oxidative damage. (8) Thioredoxin: A protein that reduces oxidized proteins, maintaining cellular redox balance. These antioxidants work synergistically to maintain cellular health and protect against oxidative damage.
(D) Here are some established nonenzymatic sources of antioxidants: (1) Vitamin C (Ascorbic Acid): Found in citrus fruits, berries, and green vegetables, it helps neutralize free radicals and regenerate other antioxidants. (2) Vitamin E (Tocopherol): Present in nuts, seeds, and vegetable oils, it protects cell membranes from oxidative damage. (3) Carotenoids: These include beta-carotene, lycopene, and lutein, found in carrots, tomatoes, and leafy greens, which help protect cells from damage. (4) Flavonoids: Found in fruits, vegetables, tea, and wine, they have strong antioxidant properties. (5) Glutathione: Although it can also be enzymatic, it acts as a nonenzymatic antioxidant in certain contexts. (6) Uric Acid: A product of purine metabolism, it has antioxidant properties. (7) Melatonin: Known for regulating sleep, it also has antioxidant properties. (8) Polyphenols: Found in fruits, vegetables, tea, and coffee, they have strong antioxidant effects. (9) Curcumin: The active compound in turmeric, known for its anti-inflammatory and antioxidant properties. (10) Bilirubin: A breakdown product of heme, it has antioxidant properties. These nonenzymatic antioxidants play a crucial role in protecting cells from oxidative stress and maintaining overall health.
Epilepsy and Regression
I. Seizure, oxidation, and autoimmunity
Excessive glutamate can cause significant neuronal damage through excitotoxicity, particularly in poorly controlled epilepsy. Oxidative stress seems to play a critical role, not in seizure control, but in triggering damage following seizures. Antioxidants like vitamin E and glutathione may mitigate this damage by combating oxidative stress (see Figure 3). (Cf. previous blog entitled as: “Defining Autism Spectrum Disorders: Mechanisms of Developmental Regression in Autism”).
These inflammatory and oxidative systems are deeply interconnected, with oxidative stress potentially exacerbating inflammation and vice versa. Chronic inflammation is a significant driver in conditions like diabetes, cardiovascular disease, neurodegeneration, cancer, and even in aging. Growing evidence suggests inflammation plays a critical role in some epilepsy cases. Steroidal and anti-inflammatory drugs can be effective in drug-resistant epilepsy, hinting at an underlying immunological component.
Certain epilepsies, such as Dravet syndrome, can be triggered by immune challenges like fever or infections (see Box-1). This association further points to an intricate link between the immune system and seizure activity. Some cases of idiopathic epilepsy might be autoimmune in origin, with early research suggesting up to 25% of such cases. This opens up potential avenues for treatment with immunotherapies alongside traditional anti-seizure medications.
These salient points underscore the complex and multifaceted nature of epilepsy and its potential connections to broader physiological systems. It’s clear that a holistic approach, considering both neurochemical and immune factors, is crucial in understanding and treating epilepsy.
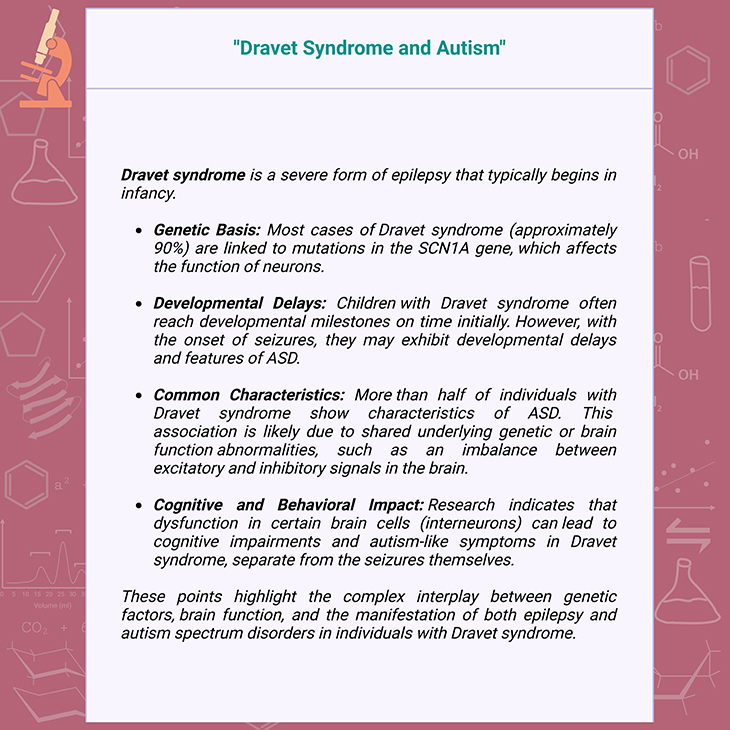
Box-1. Dravet syndrome and autism.
II. Epilepsy-induced regression
The relationship between epilepsy and autistic regression in fact involves many complexities and nuances. Epileptic encephalopathies, such as Landau-Kleffner syndrome, are prime examples where seizure activity leads to cognitive and language decline, resembling autism (see Box-2) [6]. This has spurred significant interest and research into the potential links between seizures and autistic regression. However, the findings are mixed. Some studies suggest a connection between epileptiform activity and regression, while others do not find a significant association. This inconsistency may stem from differences in study methodologies, definitions of regression, and patient demographics.
Uncontrolled seizures can cause neuronal damage, and current research indicates that autistic regression might be linked to a loss in neuronal connectivity (see Figure 4). The language regression seen in Landau-Kleffner syndrome is similar to that in idiopathic autism, suggesting that epilepsy could trigger regression in some autism cases. Yet, the prevalence and exact mechanisms remain uncertain, necessitating further research to clarify these relationships and improve treatment strategies.
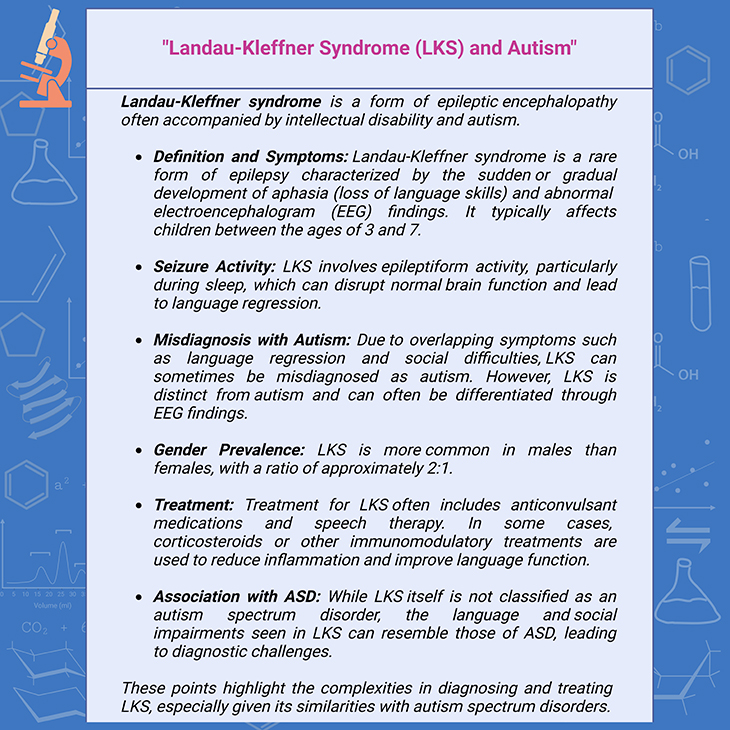
Box-2. Landau-Kleffner syndrome (LKS) and autism.
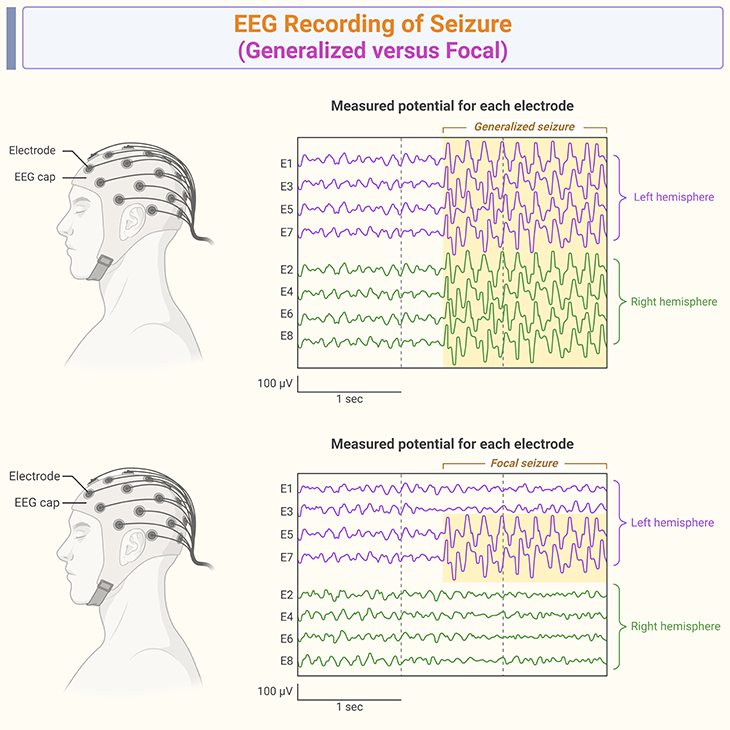
Figure 4. EEG recording of seizure. Electroencephalogram (EEG) recordings of epilepsy-induced regression in autism: Studies have shown that children with autism spectrum disorder (ASD) have higher rates of epilepsy compared to the general population. The prevalence of epilepsy in children with ASD ranges from 5% to 46%. Epileptiform EEG abnormalities, such as spikes and sharp waves, are more common in children with ASD and epilepsy. These abnormalities can indicate a higher risk of seizures and neurodevelopmental regression. Epilepsy is significantly associated with autistic regression. Children with epilepsy are more likely to experience regression in language and social skills compared to those without epilepsy. Epileptiform EEG abnormalities during the first year of life are associated with abnormal development and an increased risk of autistic regression. The comorbidity of epilepsy and ASD can complicate the clinical picture, making it essential to monitor EEG activity in children with ASD for early detection of epileptiform activity and potential regression . These facts highlight the importance of EEG monitoring in children with ASD to detect and manage epilepsy, which can contribute to neurodevelopmental regression.
[https://www.frontiersin.org/journals/psychiatry/articles/10.3389/fpsyt.2021.686021/full?formCode=MG0AV3; https://link.springer.com/article/10.1007/s00787-004-0353-7?formCode=MG0AV3;
https://www.nature.com/articles/pr2009132.pdf?formCode=MG0AV3]
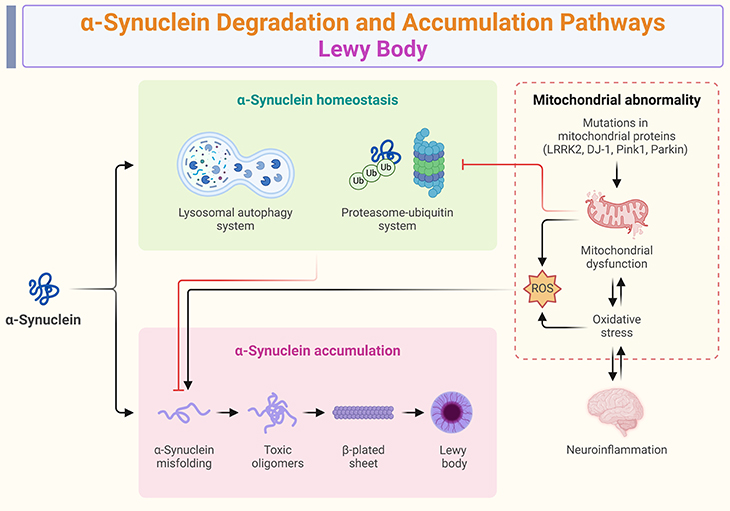
Figure 5. Alpha-synuclein degradation and accumulation pathways ~ Lewy body diseases. Alpha-synuclein is a protein that, when misfolded, forms aggregates known as Lewy bodies, which are characteristic of several neurodegenerative disorders, including Parkinson’s disease and dementia with Lewy bodies. Normally, α-synuclein is degraded by autophagy, a cellular process that breaks down and recycles damaged or unnecessary cellular components. Impairment in autophagy can lead to the accumulation of α-synuclein. α-synuclein is also degraded by lysosomes, which are cellular organelles containing enzymes that break down waste materials. Dysfunction in lysosomal pathways can contribute to the buildup of α-synuclein. Misfolded alpha-synuclein proteins aggregate into insoluble intracellular deposits called Lewy bodies. These aggregates are believed to disrupt normal cellular functions and contribute to neurodegeneration. Mutations and multiplication of the SNCA gene, which encodes α-synuclein, have been linked to familial Parkinson’s disease. Increased levels of α-synuclein due to these genetic alterations can promote aggregation and Lewy body formation. Some studies suggest that the early loss of normal, functional α-synuclein might be a major driver of neurodegeneration, rather than just the accumulation of misfolded protein. These pathways and mechanisms highlight the complex interplay between protein degradation, aggregation, and genetic factors in the development of Lewy body diseases.
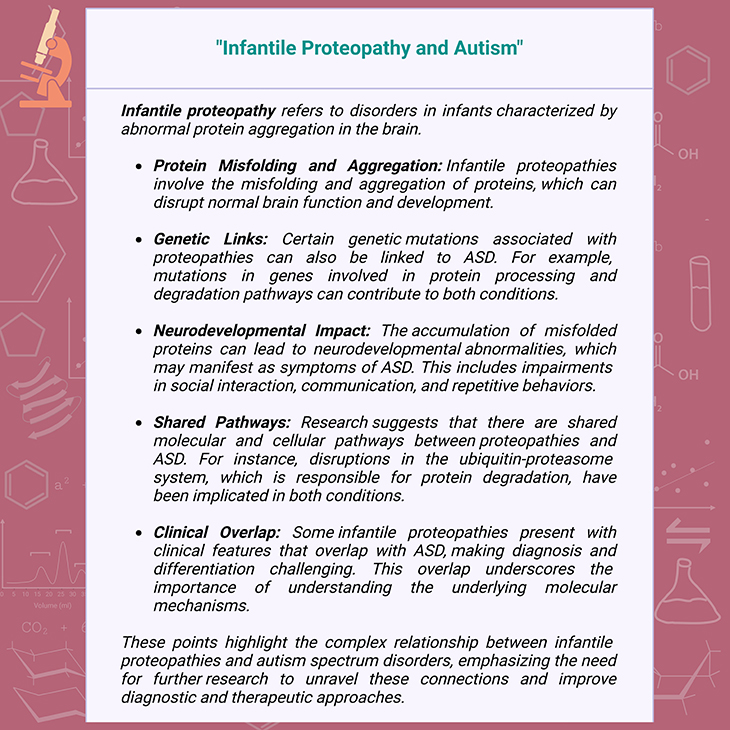
Box-3. Infantile proteopathy and autism.
Infantile Proteopathy and Tauopathy
I. Infantile Proteopathy
What is Proteopathy? Proteopathy is a condition that involves the misfolding and aggregation of proteins, leading to cellular stress, damage, and ultimately, cell death (see Figure 5; Box-3). Classic examples include some of the adult and senile neurodegenerative conditions, such as:
- Alzheimer’s disease – with its characteristic Tau protein neurofibrillary tangles and plaques;
- Lewy body dementia – with its Lewy bodies composed of misfold α-synuclein protein; and
- Huntington’s disease – in which proteins produced from the mutated HTT gene contain expanded number of amino acids glutamine, which eventually leads to misfolding and aggregation of the Huntingtin protein.
Unlike adult neurodegenerative conditions, infantile proteopathies may not cause obvious tissue damage due to the biological plasticity of youth. Instead, they impair cell function, affecting development and potentially leading to intellectual disabilities and developmental delay.
One type of infantile proteopathy – particularly a tauopathy – with overlap with autism spectrum disorders is tuberous sclerosis (TSC). TSC is a genetic disorder caused by mutations in the TSC1 or TSC2 genes, leading to benign tumor growth in various organs, including skin, brain, kidneys, heart, and lungs.
TSC occurs in approximately 5 in 10,000 births. TSC2 mutations are more common and are associated with a higher prevalence of autism (~40%) compared to TSC1 mutations (M~3%). Around 75% of cases of autism associated with TSC are due to TSC2 mutations. This highlights the genetic link between proteopathies and neurodevelopmental disorders like autism (see Figure 5; Box-4) [7].
These key facts underscore the significance of understanding proteopathies beyond adult neurodegenerative diseases, emphasizing their impact on early development and their association with conditions like ASD.
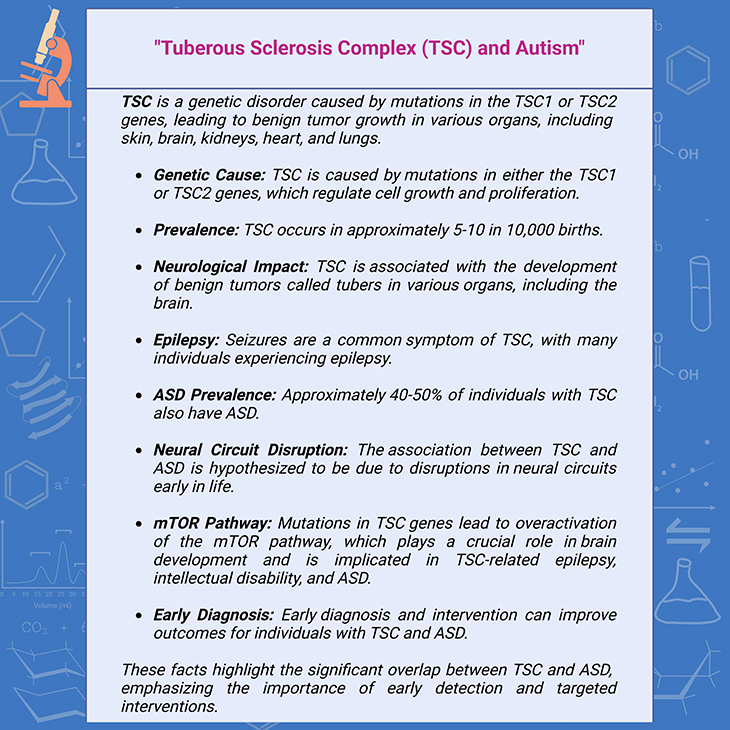
Box-4. Tuberous sclerosis complex (TSC) and autism.
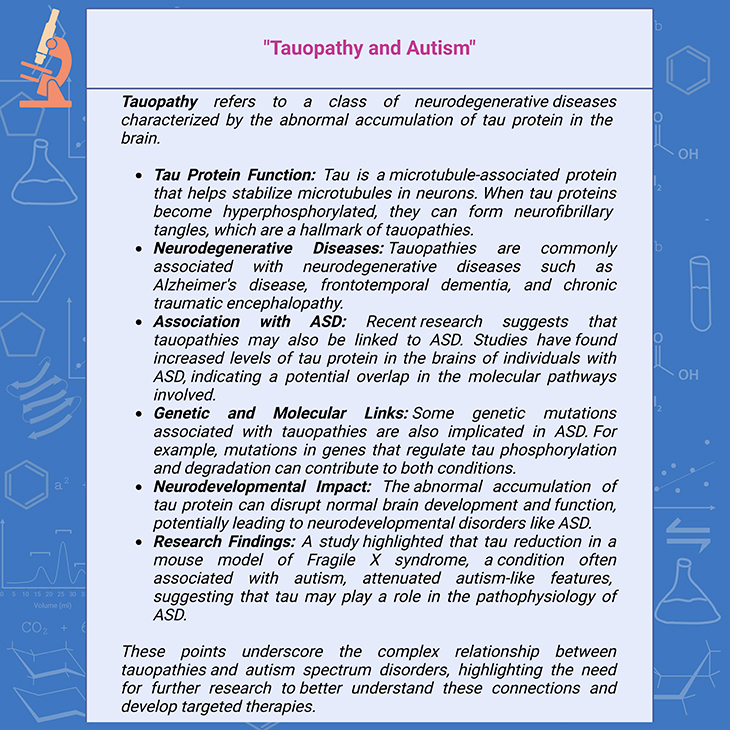
Box-5. Tauopathy and autism.
II. Tauopathies
Down syndrome, like tuberous sclerosis, also shares significant comorbidity with ASD. Approximately 13% of individuals with Down syndrome also have autism. Unfortunately, many individuals with Down syndrome develop Alzheimer’s disease, with a prevalence of 40% by ages 50-59. Both Down syndrome and Alzheimer’s disease are considered tauopathies, characterized by neurofibrillary tangles and plaques in the brain (see Figure 5; Box -5; Box-6) [8 -9]].
Around 50% of children with Down syndrome and comorbid autism experience regression in language or socio-communicative skills, typically occurring in late childhood. Given the tauopathy nature of Down syndrome, it’s hypothesized that tau pathology could contribute to autistic regression in these individuals. This raises the possibility that other forms of autistic regression might also result from infantile proteopathies; and underscoring the complex interactions between genetic syndromes, neurodevelopmental disorders, and neurodegenerative diseases. The overlapping characteristics of these conditions signifying the need for further research to unravel these connections and develop effective treatments.
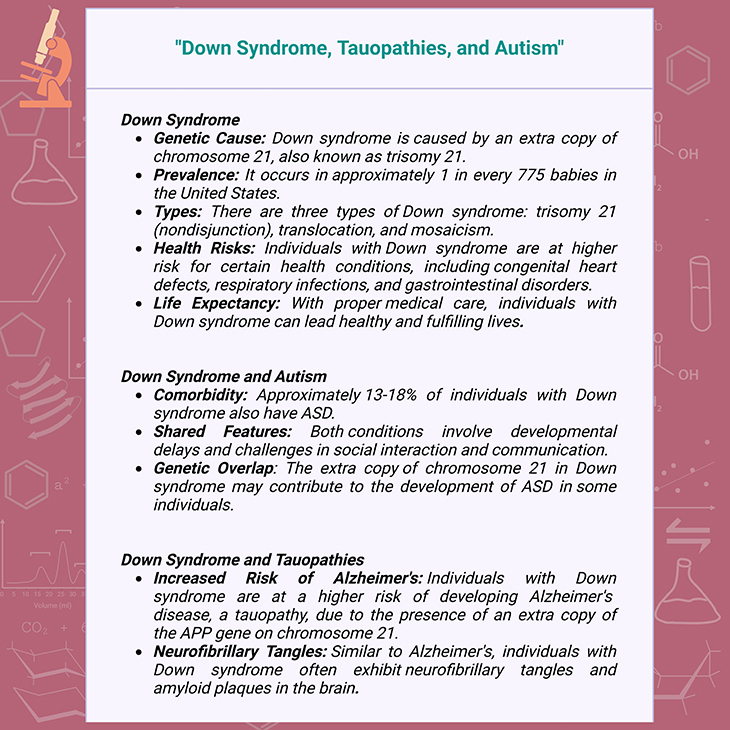
Box-6. Down syndrome, tauopathies, and autism.
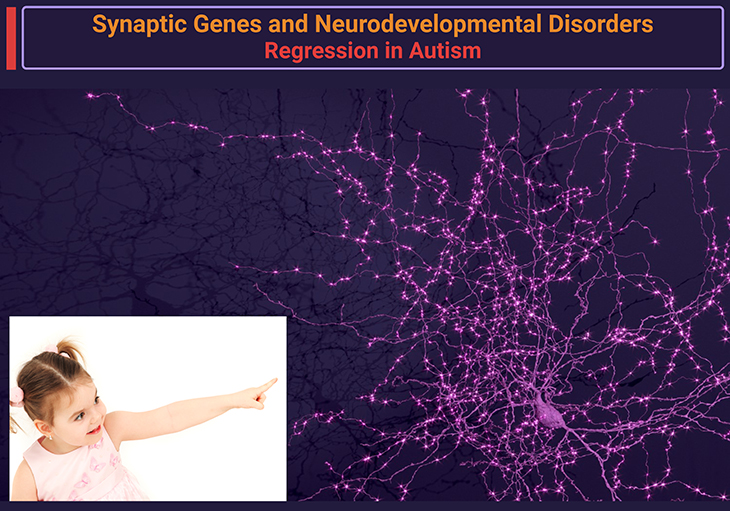
Figure 6. Synaptic genes and regression in autism. Synaptic genes are crucial for the development and function of synapses, which are the connections between neurons. Mutations in synaptic genes have been linked to autism, particularly in cases where children experience regression. Research has shown that children with ASD and mutations in postsynaptic density genes are more likely to experience regression. Changes in the expression of synaptic genes in the brains of individuals with ASD may play a causal role in the development of the disorder. Specific synaptic genes that have been associated with regression in autism include, for example, NRXN1 (Neurexin 1), NLGN3 (Neuroligin 3), SHANK3 (SH3 and multiple ankyrin repeat domains 3), TSC1 (Tuberous Sclerosis 1), TSC2 (Tuberous Sclerosis 2), FMR1 (Fragile X Mental Retardation 1), MECP2 (Methyl CpG Binding Protein 2). These genes play important roles in synaptic function and have been linked to autism, particularly in cases involving regression [10]. [https://pubmed.ncbi.nlm.nih.gov/26289574/] {Image credit – modified and adapted from www.microns-explorer.org}
Central Role of Synaptic Health
I. Synaptic dysfunction and neurodegenerative diseases
- Tau and Synapses: Research indicates that memory impairment in neurodegenerative diseases, like Alzheimer’s, can begin with synaptic dysfunction long before the formation of neurofibrillary tangles and plaques.
- Alpha-Synuclein: This protein, implicated in Lewy body dementia, Parkinson’s, and some forms of Alzheimer’s, is highly expressed at the synapse and may play a role in neurotransmitter release and synaptic plasticity.
- Huntingtin Protein: Similar to Tau and alpha-synuclein, the Huntingtin protein, responsible for Huntington’s disease, is also enriched at the synapse. Early disease pathology includes synaptic impairment.
II. Synaptic genes and neurodevelopmental disorders
- Genetic Links: Recent studies suggest that autistic children with mutations in synaptic genes are more likely to experience regression compared to those with other types of mutations.
- Syndromic Autism Features: Syndromic forms of autism, such as those associated with specific genetic conditions, often exhibit neurodegenerative-like features, including brain atrophy and psychomotor dysfunction like spasticity and hyperreflexia (overactive reflexes).
Taken together, these studies provide early yet promising evidence that synaptic dysfunction may be a key player in autistic regression. Meanwhile, epilepsies, illnesses, autoimmunity, proteopathies, and oxidative stress can all converge on and exacerbate synaptic vulnerabilities, potentially leading to neurodevelopmental and neurodegenerative conditions (see Figure 5; Figure 6; Box-5; Box-6).
These points underscore the central role of synaptic health in both neurodevelopmental and neurodegenerative diseases. Understanding these connections could be crucial for developing targeted therapies that address synaptic dysfunction. Fascinating stuff, right? It’s like uncovering the intricate threads of a very complex tapestry.
Clinical Considerations
In this section, we would like to emphasize some crucial points about the complexities of managing autistic regression, especially in relation to immune challenges and vaccination schedules. Here are some established facts and considerations:
1. Underlying causes of autistic regression
- Genetic factors: Mutations in synaptic genes can increase the likelihood of autistic regression.
- Immune challenges: Both metabolic and immunological disorders can trigger regression, often through immune challenges such as infections or vaccinations.
- Metabolic disorders: Some metabolic disorders may not become apparent until regression onset.
2. Vaccination schedules and adverse reactions
- Later schedules: Delaying vaccination schedules can reduce the likelihood of adverse reactions.
- Combining vaccines: Combining multiple vaccines can modestly increase the risk of adverse reactions, suggesting that spacing them out may reduce risk.
- Case-by-case basis: If a child experiences a significant adverse reaction, healthcare providers may consider altering or discontinuing the vaccine schedule.
3. Healthcare Provider Recommendations
- Full history: Doctors should take a comprehensive history of the child and their first-degree relatives to identify potential immune or metabolic dysregulation.
- Significant reactions: Distinguishing between significant adverse reactions and typical mild responses is crucial.
- Precautionary measures: Taking these precautions can help reduce serious adverse reactions while maintaining protection against infections.
These points underscore the importance of personalized medical care and the need for further research into the etiologies of regression and heritable factors. It is a delicate balance between protecting children from infections and managing potential risks associated with vaccinations.
Take Home Messages
Epilepsy and Autism
- Epileptic encephalopathies: Conditions like Landau-Kleffner syndrome highlight how seizure activity can lead to cognitive and language decline.
- Neuronal connectivity: Uncontrolled seizures can disrupt neuronal connectivity, potentially triggering autistic regression.
- Inflammation: Immune challenges often play a crucial role in triggering regression in conditions like Dravet syndrome.
Down Syndrome and Neurodegeneration
- Comorbidity with ASD: Approximately 13% of individuals with Down syndrome also have autism.
- Risk of Alzheimer’s: Individuals with Down syndrome are at a higher risk of developing Alzheimer’s disease due to tauopathy.
- Synaptic dysfunction: Synaptic impairment is a significant factor in neurodegenerative diseases and may also play a role in autistic regression.
Proteopathies and Neurodevelopment
- Infantile proteopathies: Conditions like tuberous sclerosis demonstrate how protein misfolding can impact early brain development and lead to intellectual disabilities and autism.
- Synaptic genes: Mutations in synaptic genes are more likely to be associated with autistic regression.
Inflammation, Oxidation, and Autoimmunity
- Excitotoxicity: Excessive glutamate can cause neuronal damage in epilepsy.
- Oxidative stress: Chronic inflammation and oxidative stress are linked to various diseases, including neurodegeneration and diabetes.
- Autoimmune epilepsy: Some cases of epilepsy may have an autoimmune component, highlighting the need for personalized treatment approaches.
Vaccination and Immune Challenges
- Vaccination schedules: Delaying or spacing out vaccinations may reduce adverse reactions, especially in children with a family history of immune or metabolic disorders.
- Case-by-case basis: Healthcare providers should consider individual and familial health histories when determining vaccination schedules.
Overall Message
- Complex interactions: The interplay between genetic factors, immune responses, and environmental triggers is crucial in understanding and managing neurodevelopmental and neurodegenerative conditions.
- Personalized approaches: Tailored interventions based on individual and familial health profiles can improve outcomes in conditions like autism and epilepsy.
These takeaways highlight the intricate and interconnected nature of these conditions and underscore the importance of continued research and personalized healthcare.
Conclusions
Understanding the complex interplay between genetic factors, immune responses, and environmental triggers is crucial for managing neurodevelopmental and neurodegenerative conditions. Personalized approaches based on individual and familial health profiles can improve outcomes in conditions like autism and epilepsy. Continued research is essential to uncover the underlying mechanisms and develop targeted therapies.
For information on autism monitoring, screening and testing please read our blog.
References
- Yao Y, Xu XH, Jin L. Macrophage Polarization in Physiological and Pathological Pregnancy. Front Immunol. 2019 Apr 15;10:792. doi: 10.3389/fimmu.2019.00792. PMID: 31037072; PMCID: PMC6476302.
https://pubmed.ncbi.nlm.nih.gov/31037072/ - Singer HS, Morris CM, Williams PN, Yoon DY, Hong JJ, Zimmerman AW. Antibrain antibodies in children with autism and their unaffected siblings. J Neuroimmunol. 2006 Sep;178(1-2):149-55. doi: 10.1016/j.jneuroim.2006.05.025. Epub 2006 Jul 13. PMID: 16842863.
https://pubmed.ncbi.nlm.nih.gov/16842863/ - Matelski L, Van de Water J. Risk factors in autism: Thinking outside the brain. J Autoimmun. 2016 Feb;67:1-7. doi: 10.1016/j.jaut.2015.11.003. Epub 2015 Dec 22. PMID: 26725748; PMCID: PMC5467975.
https://pubmed.ncbi.nlm.nih.gov/26725748/ - Cunningham C. Microglia and neurodegeneration: the role of systemic inflammation. Glia. 2013 Jan;61(1):71-90. doi: 10.1002/glia.22350. Epub 2012 Jun 6. PMID: 22674585.
https://pubmed.ncbi.nlm.nih.gov/22674585/
- Li BM, Liu XR, Yi YH, Deng YH, Su T, Zou X, Liao WP. Autism in Dravet syndrome: prevalence, features, and relationship to the clinical characteristics of epilepsy and mental retardation. Epilepsy Behav. 2011 Jul;21(3):291-5. doi: 10.1016/j.yebeh.2011.04.060. Epub 2011 May 26. PMID: 21620773.
https://pubmed.ncbi.nlm.nih.gov/21620773/
- Zafari A, Karimi N, Taherian M, Taherian R. Landau Kleffner Syndrome and Misdiagnosis of Autism Spectrum Disorder: A Mini-Review. Int Clin Neurosci J [Internet]. 2018 Mar. 15 [cited 2024 Oct. 6];5(1):3-6.
https://journals.sbmu.ac.ir/neuroscience/article/view/19459 - Numis AL, Major P, Montenegro MA, Muzykewicz DA, Pulsifer MB, Thiele EA. Identification of risk factors for autism spectrum disorders in tuberous sclerosis complex. Neurology. 2011 Mar 15;76(11):981-7. doi: 10.1212/WNL.0b013e3182104347. PMID: 21403110; PMCID: PMC3271577.
https://pubmed.ncbi.nlm.nih.gov/21403110/ - Krüger L, Mandelkow EM. Tau neurotoxicity and rescue in animal models of human Tauopathies. Curr Opin Neurobiol. 2016 Feb;36:52-8. doi: 10.1016/j.conb.2015.09.004. Epub 2015 Sep 29. PMID: 26431808.
https://pubmed.ncbi.nlm.nih.gov/26431808/
- Fuster-Matanzo A, Hernández F, Ávila J. Tau Spreading Mechanisms; Implications for Dysfunctional Tauopathies. Int J Mol Sci. 2018 Feb 25;19(3):645. doi: 10.3390/ijms19030645. PMID: 29495325; PMCID: PMC5877506.
https://pubmed.ncbi.nlm.nih.gov/29495325/
- Bourgeron T. From the genetic architecture to synaptic plasticity in autism spectrum disorder. Nat Rev Neurosci. 2015 Sep;16(9):551-63. doi: 10.1038/nrn3992. PMID: 26289574.
https://pubmed.ncbi.nlm.nih.gov/26289574/