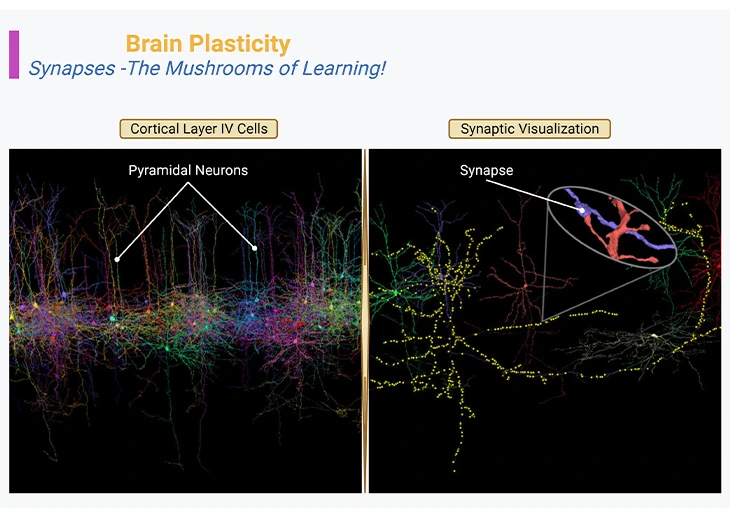
Figure 1: Brain Plasticity – Synapses – The Mushrooms of Learning. Neocortex layer IV, the internal granular layer, consisting of pyramidal neurons or cells (left panel). Three-dimensional reconstruction of the synapses that connect the axon of one neuron [purple blue] with the dendrites of another neuron [orange red] (right panel, inset).
{Image credits – modified and adapted from: www.microns-explorer.org}.
Introduction
When we discuss about brain plasticity, the term must be taken in a very literal sense, i.e., ‘brain is a pliable organ! ’
Brain Plasticity: What It is
Brain plasticity, also referred to as neural plasticity, neuroplasticity, cortical plasticity, or brain remapping. Neural plasticity is defined as “the changes in neural organization which may account for various forms of behavioral modifiability, either short-lasting or enduring, including maturation, adaptation to a mutable environment, specific and unspecific kinds of learning, and compensatory adjustments in response to functional losses from ageing or brain damage” [1].
Synapses: The Mushrooms of Learning
With the arrival and invention of next generation of microscopes, it has become possible to visualize not only the anatomy of synapses, but also their dynamics in real time. And as odd as it may seem, neurons constantly move, especially during postnatal development and education. In general, their axonal tips grow and shrink as they grope around for new contacts or connections, while their dendritic trees bear the birth and death of new synapses, with a turnover of millions of synapses per second in the developing child’s brain (Figure 1).
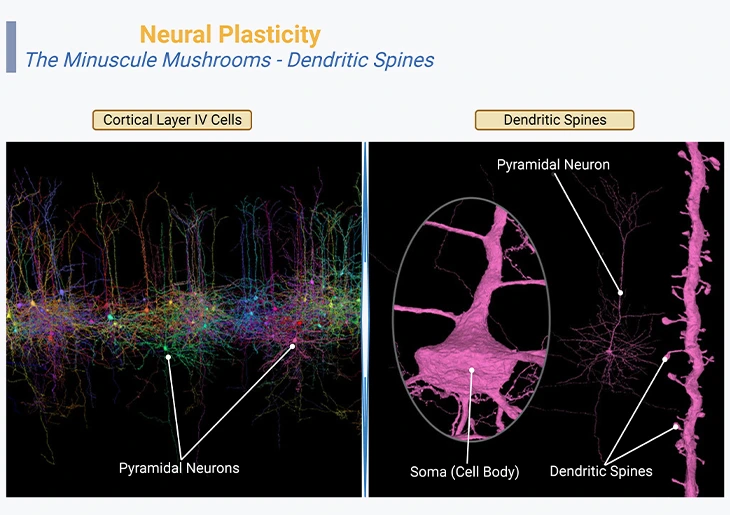
Figure 2: Neural Plasticity – The Minuscule Mushrooms – Dendritic Spines. Neocortex layer IV, the internal granular layer, consisting of pyramidal neurons or cells (left panel). Three-dimensional reconstruction of the pyramidal cell dendritic spines and body [fuchsia] (right panel, inset).
{Image credits – modified and adapted from: www.microns-explorer.org}.
Dendritic Spines: The Minuscule Mushrooms
The tiny or minuscule mushroom-like structures that cover the branches of this neuron are called “dendritic spines. ” Their spiny or bristly shapes are characteristics of pyramidal neurons of the cerebral cortex, the hippocampus, and the amygdala. Each spine hosts one or at times several synapses coming in from other neighboring neurons. These organelles are highly dynamic structures, ceaselessly moving, and in reality, their size and even their very existence can change within a few tens of minutes as a function of learning (Figure 2).
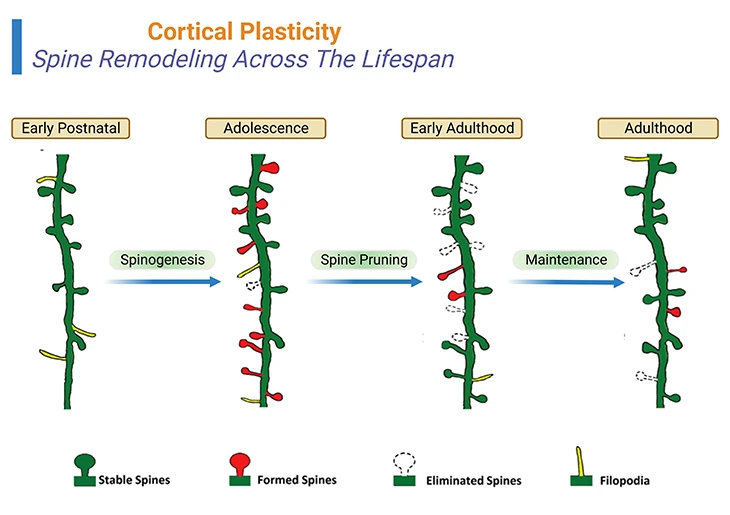
Figure 3: Cortical Plasticity – Spine Remodeling Across The Lifespan: Spine remodeling across the life span of a human being. Rapid spinogenesis in early postnatal is proceeded by a gradual spine pruning in adolescence. In adulthood, spine formation and elimination reach equilibrium, with a minor fraction of spines constantly added or removed. However, experience affects spine dynamics differently at different developmental stages. {Image credits – modified and adapted from: Chen CC, et al., (2014) Front Neuroanat. 8: 28.} [2].
Neuronal activity modulates the strength and stability of synapses. At the very least, a synapse whose two neurons (the pre-synaptic and post-synaptic) discharge simultaneously increases in strength, i.e., “neurons that fire together wire together.” In this scenario, the size of the synapse grows to accommodate more receptor molecules. Its strength is directly reflected in the size of the spine’s neck, which increases consequently. In contrast, if a synapse is no longer useful, and if it goes against the flow, then its effectiveness decreases, and it may eventually retract and vanish [3].
Spine Dynamics: Spine Remodeling Across Lifespan
Rapid spinogenesis in early postnatal is followed by a gradual spine pruning in adolescence. In adulthood, spine formation and elimination reach equilibrium, with a minor fraction of spines constantly added or removed. However, experience affects spine dynamics differently at different developmental stages [2] (Figure 3; Box-1).
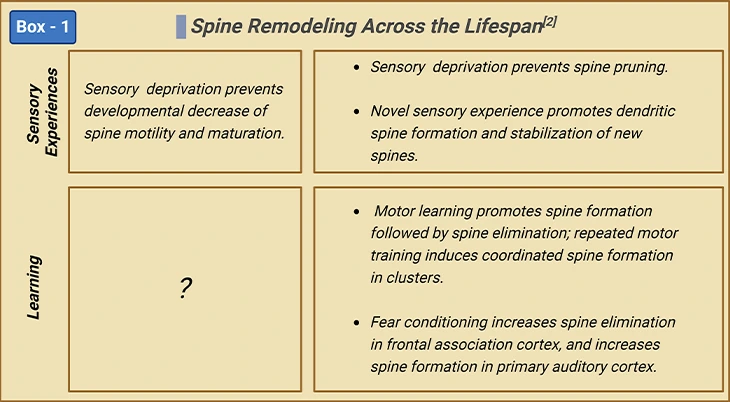
Artificial and Biological Neural Networks
Neural networks, also known as artificial neural networks (ANNs) or simulated neural networks (SNNs), are a subset of machine learning and are at the heart of deep learning algorithms. And they are inspired by the human brain, and consequently mimicking the way that biological neurons signals to one another.
ANNs help us understand how millions of synaptic changes allow us to learn and recognize, for example, a face or an object. In the real brain, however, synapses are not the only correlate of learning. Axons, and especially their levels of myelination, may also change in order to accelerate the process of information.
In the cerebellum, learning may also be based on adjustments purely internal to the cell, without any synaptic modification. The conventional view of neural signaling is that a neuron (pre-synaptic) can influence its target cell (post-synaptic) by exciting or inhibiting it. A significant aspect of the classical view is that learning consists of modifying the efficacy of synapses, i.e., either strengthening (long-term potentiation, LTP) or weakening (long-term depression, LTD) them. However, in studying how cerebellar Purkinje cells change their responsiveness to a particular stimulus during learning of conditioned responses, it has been shown that these cells can learn the temporal relationship between two paired stimuli. The cells learn to respond at a particular time that reflects the time between the stimuli. This critical finding radically changes our current view of both neural signaling and learning [4]. Nevertheless, we are still far from the understanding the vast nanometer-scale flexibility of the developing brain.
Conclusions
Understanding how the spine dynamics correlate with anatomical and physiological features of specific neural circuits in different behavioral context is crucial to the elucidation of the information processing and storage mechanism in the brain. Several studies over the last two decades have demonstrated that learning is essentially based on either the strengthening or the elimination of synapses, which represents the memory traces of our experiences.
For information on autism monitoring, screening and testing please read our blog.
References
- Berlucchi G, Buchtel HA. Neuronal plasticity: historical roots and evolution of meaning. Exp Brain Res. 2009 Jan;192(3):307-19. doi: 10.1007/s00221-008-1611-6. Epub 2008 Nov 12. PMID: 19002678.
https://pubmed.ncbi.nlm.nih.gov/19002678/ - Chen CC, Lu J, Zuo Y. Spatiotemporal dynamics of dendritic spines in the living brain. Front Neuroanat. 2014 May 9;8:28. doi: 10.3389/fnana.2014.00028. PMID: 24847214; PMCID: PMC4023020.
https://pubmed.ncbi.nlm.nih.gov/24847214/ - Grutzendler J, Kasthuri N, Gan WB. Long-term dendritic spine stability in the adult cortex. Nature. 2002 Dec 19-26;420(6917):812-6. doi: 10.1038/nature01276. PMID: 12490949.
https://pubmed.ncbi.nlm.nih.gov/12490949/ - Johansson F, Jirenhed DA, Rasmussen A, Zucca R, Hesslow G. Memory trace and timing mechanism localized to cerebellar Purkinje cells. Proc Natl Acad Sci U S A. 2014 Oct 14;111(41):14930-4. doi: 10.1073/pnas.1415371111. Epub 2014 Sep 29. PMID: 25267641; PMCID: PMC4205653.
https://pubmed.ncbi.nlm.nih.gov/25267641/