Table of Contents
- Introduction
- Flames of Life: How Combustion Shapes Our Vital Energy
- Breathing in Color: The Evolution of Cellular Respiration
- The Power Relay: How the Respiratory Chain Fuels Life
- Take-Home Messages
- Summary and Conclusions
- Did You Know About Folate Receptor Autoantibodies (FRAAs) and Brain Development?
- References
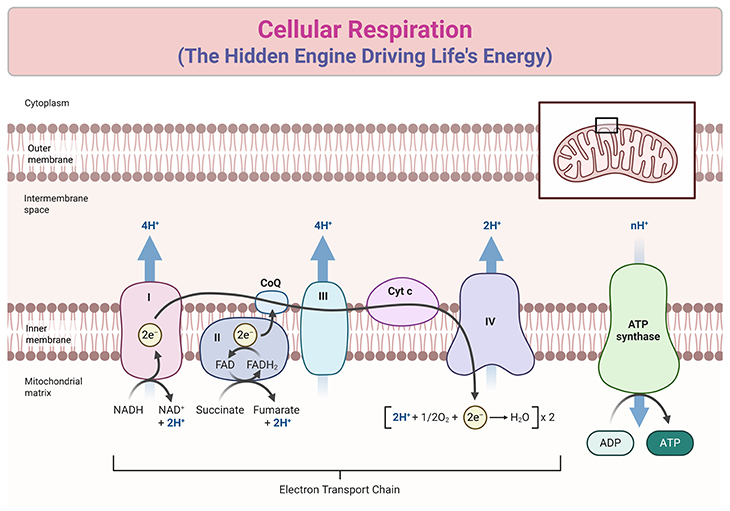
Figure 1. The Respiratory Chain: A Molecular Circuit of Energy Transformation. A simplified diagram of the respiratory chain illustrates the roles of Complexes I, II, III, and IV, along with the ATPase (Complex V). Electrons (e⁻) enter the chain via either Complex I or Complex II and are subsequently transferred to Complex III by ubiquinone (Coenzyme Q, CoQ), a mobile carrier often marketed as a health supplement despite its uncertain efficacy. The electron flow is depicted as a curving line through the chain. From Complex III, electrons are shuttled to Complex IV (cytochrome oxidase) by cytochrome c (Cyt c). At Complex IV, electrons combine with protons and oxygen to form water. All the complexes are shown embedded as distinct entities within the membrane. While ubiquinone and cytochrome c facilitate electron transfer between complexes, the critical link connecting electron transport to ATP synthesis via the ATPase remained a perplexing mystery for many years.
Introduction
The Essence of Cellular Respiration: Respiration is the cornerstone of life, a biochemical process that converts the energy stored in food molecules into a usable form, empowering every cellular function. At its core, respiration involves the oxidation of glucose, where electrons and protons are extracted and systematically transferred through a series of reactions. These stepwise transfers not only prevent energy from being wasted in uncontrolled bursts but also enable its conservation for vital processes like muscle contraction, neural activity, and cellular maintenance.
Central to this process is the respiratory chain—a meticulously organized sequence of redox reactions within the mitochondria. Electrons are passed along a chain of molecular complexes embedded in the inner mitochondrial membrane, releasing energy at each step. This energy powers the production of adenosine triphosphate (ATP), the universal energy currency of life. Accompanied by the controlled movement of protons, this carefully orchestrated flow is what fuels cellular work (see Figure 1).
The key players in respiration include glucose, oxygen, and specialized molecules like cytochromes and coenzymes. Together, these components create a molecular relay system that transforms chemical potential energy into a form that powers diverse cellular activities. By linking the breakdown of glucose to the precise release and conservation of energy, respiration ensures the continuity of life’s intricate processes.
This article unpacks the intricate mechanisms underlying respiration, including its pivotal molecular players, the organization of the respiratory chain, and how this process is the ultimate engine of energy transformation within living cells.
Flames of Life: How Combustion Shapes Our Vital Energy
The Spark of Life: From Poetry to Science: For centuries, poets and philosophers waxed lyrical about the “flame of life,” a metaphor for human vitality. Paracelsus, a 16th-century alchemist, likened human survival to a fire extinguished without air. But in the late 18th century, the groundbreaking chemist Antoine Lavoisier shattered the poetic veil with a radical notion: life’s flame is no metaphor—it is literal combustion. Lavoisier’s research revealed that respiration is, at its core, a slow-burning reaction akin to the flickering of a candle. This biological combustion, he argued, is powered by carbon and hydrogen from food, with the blood acting as a conveyor of fuel. Like a lamp that burns oil, animals burn nutrients to sustain life, though they must replenish their reserves through food (see Box-1). Tragically, Lavoisier’s revolutionary insights were cut short by the guillotine during the French Revolution.
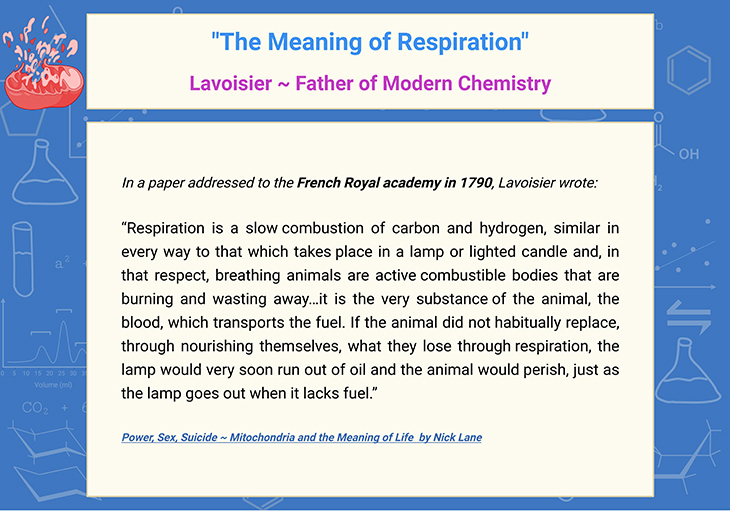
Box-1. The Meaning of Respiration: Antoine Lavoisier ~ Father of Modern Chemistry.
Homing In: The Hunt for Respiration’s Seat: Lavoisier’s ideas redefined our understanding of respiration, yet his guesses about where it occurred were off the mark. He believed that combustion unfolded in the blood as it passed through the lungs. The true location of respiration remained a mystery for nearly a century, igniting fierce debates. It was not until 1870 that Eduard Pflüger proved that respiration happens within the individual cells of all living organisms. Even then, scientists mistakenly pinpointed the nucleus as the site of activity. Only in 1912 did B.F. Kingsbury propose mitochondria as the true hub of respiration, a theory confirmed decades later in 1949 when researchers Eugene Kennedy and Albert Lehninger identified respiratory enzymes within these cellular powerhouses [1-4].
The Chemistry of Combustion: Oxidation Unveiled: Respiration’s central process, the oxidation of glucose, is an electrochemical marvel. At its heart, oxidation involves losing electrons, while reduction involves gaining them. In respiration, oxygen acts as a voracious oxidizer, snatching electrons from glucose molecules. These electrons, coupled with protons, transform oxygen into water—a vital byproduct of this energy-releasing process (see Figure 1). Such oxidation and reduction (redox) reactions are fundamental to life, driving energy production in cells. Remarkably, redox reactions are not unique to oxygen-based systems; even a battery operates on similar principles, with electrons flowing from a source to an acceptor.
Beyond Combustion: The Energy Conundrum: While Lavoisier brilliantly linked respiration to combustion, he misinterpreted its ultimate purpose, believing it solely generated heat—a view rooted in the scientific limitations of his time. By the mid-19th century, the dawn of thermodynamics offered a clearer picture of energy transformation. Pioneers like James Prescott Joule and Lord Kelvin demonstrated that heat and mechanical work are interchangeable, leading to the first law of thermodynamics: energy cannot be created or destroyed, only transformed. German physicist Hermann von Helmholtz extended these principles to biology, revealing that the energy released from food fuels not only heat but also mechanical work, like muscle contraction.
Unlocking Molecular Potential: Energy for Life’s Work: The modern understanding of energy has illuminated how living organisms harness the potential energy stored in molecular bonds. When glucose combusts during respiration, its energy is not wasted as heat but instead channeled into biological “currencies” like ATP (adenosine triphosphate). ATP fuels all of life’s tasks, from muscle movement to cognitive function, ensuring that every ounce of energy is converted into meaningful work. This realization marks the crux of respiration’s role—not just as a fire that burns, but as a finely-tuned system that powers the machinery of life [5-6].
This narrative bridges historical discoveries and modern science, bringing to life the intricate dance of chemistry, biology, and energy that sustains us [1-6].
Breathing in Color: The Evolution of Cellular Respiration
The Mystery of Cellular Energy Unveiled: By the late 19th century, scientists were certain that respiration occurred within cells and powered every facet of life. Yet, the mechanism behind this energy transfer was shrouded in mystery. How did the energy from glucose oxidation fuel life’s demands? The answers lay buried in the intricate dance of molecules.
Oxygen: Powerful but Picky: Glucose does not burst into flames just because oxygen is present. This is due to oxygen’s unique nature: it is thermodynamically reactive but kinetically stable, meaning it doesn’t react quickly unless activated. Activation can occur through energy input, like striking a match, or through catalysts that lower the energy needed for reactions. Victorian scientists hypothesized that iron, known for its oxygen-binding properties, played a catalytic role in respiration. Hemoglobin, the iron-rich pigment responsible for blood’s color, emerged as a potential candidate. But respiration occurs inside cells, not the bloodstream—prompting researchers to search for similar pigments within tissues.
A Tale of Pigments: The Cellular Palette: Pigments like hemoglobin reveal their secrets through their absorption spectrum—the specific wavelengths of light they absorb and transmit. Arterial blood appears red because hemoglobin absorbs blue-green and yellow light, transmitting vivid reds. Venous blood turns purple as oxygen dissociates, altering the absorption spectrum. This phenomenon inspired scientists to explore cellular pigments.
In 1884, Charles MacMunn discovered a pigment in animal tissues that he believed facilitated respiration. Yet, his findings were met with skepticism and faded into obscurity until 1925, when David Keilin rediscovered the pigment. Keilin’s groundbreaking work revealed not one but three distinct pigments, which he named cytochromes a, b, and c based on their absorption spectra—a classification still used today [2].
The Missing Link: Warburg’s Ingenious Discovery: Despite Keilin’s revelations, the cytochromes did not react directly with oxygen, leaving a critical gap in understanding. Otto Warburg, a German chemist, stepped in with an innovative approach, leveraging the chemical behavior of iron compounds exposed to carbon monoxide and light. This allowed him to infer the absorption spectrum of what he called the “respiratory ferment.” Warburg identified it as a hemin compound—similar to hemoglobin and chlorophyll—and earned the Nobel Prize in 1931 for his contributions.
Warburg’s findings suggested a fascinating evolutionary connection: the respiratory pigment, with its unique brownish hue, could transform chemically into red or green pigments resembling hemoglobin or chlorophyll. He hypothesized that respiration predates photosynthesis, implying that cellular energy processes laid the groundwork for life before the advent of plants.
The Colors of Cellular Life: The discovery of respiratory pigments was not just a scientific milestone—it was a journey into the hidden hues of life. From hemoglobin’s vibrant reds to the elusive cytochromes, these pigments hold the key to understanding how cells harness energy to sustain life. Warburg’s visionary insights bridged respiration and photosynthesis, painting a vivid picture of the molecular origins of life itself.
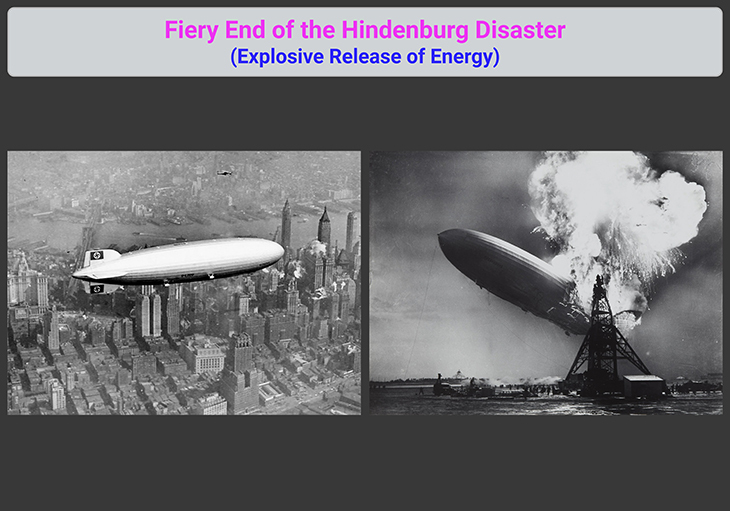
Figure 2. Hindenburg Disaster – The Lagest Zeppelin Ever Built. {Image credits – modified and adapted from: Wikipedia.} [https://en.wikipedia.org/wiki/Hindenburg_disaster]
The Power Relay: How the Respiratory Chain Fuels Life
The Chain Reaction That Powers Life: Despite landmark discoveries in cellular respiration, Otto Warburg had yet to uncover the full mechanics behind how cells harness energy. At the time of his Nobel Prize, Warburg proposed that respiration was a one-step process, where glucose released all its energy at once—a theory that failed to account for David Keilin’s discovery of cytochromes. Meanwhile, Keilin was crafting the concept of a “respiratory chain.” He envisioned glucose as a source of hydrogen atoms, stripped of their protons and electrons, then passed down a molecular chain like a relay team delivering energy to the finish line. Instead of an explosive release of energy, akin to the fiery end of the Hindenburg (see Figure 2), the chain gradually released manageable bursts, enabling this energy to be stored and directed toward work, such as muscle contraction [5-6].
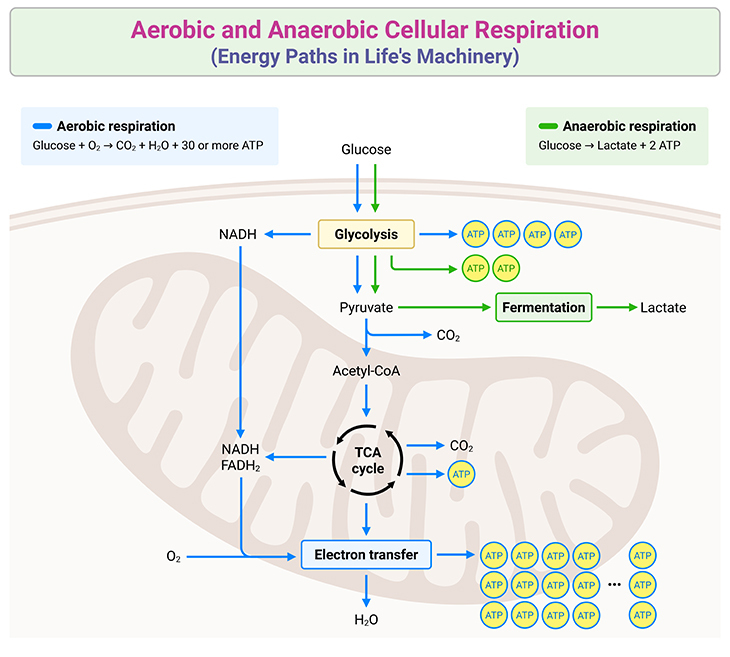
Figure 3. Aerobic and Anaerobic Cellular Respiration: Energy Paths in Life’s Machinery. Cellular respiration is the process by which cells extract energy from glucose to generate adenosine triphosphate (ATP), the universal energy currency. It occurs in two primary forms: aerobic respiration, requiring oxygen, and anaerobic respiration, which operates in oxygen-deprived environments. In aerobic respiration, glucose undergoes glycolysis in the cytoplasm, breaking down into pyruvate. Pyruvate is further oxidized in the mitochondria through the Tricarboxylic Acid (TCA) cycle, also known as the Krebs Cycle. This cycle produces high-energy electron carriers (NADH and FADH₂) that feed electrons into the respiratory chain. The electron transfer across mitochondrial complexes drives proton gradients, ultimately powering ATP synthesis via the ATPase complex. Oxygen serves as the final electron acceptor, forming water as a byproduct, while producing up to 36 ATP molecules per glucose molecule. Anaerobic respiration, on the other hand, bypasses the TCA cycle and oxidative phosphorylation. Instead, glucose is metabolized through glycolysis, yielding pyruvate. Without oxygen, pyruvate undergoes fermentation, resulting in byproducts like lactic acid in animals or ethanol and carbon dioxide in microorganisms. Anaerobic respiration produces significantly less ATP, typically only 2 molecules per glucose molecule. These complementary pathways ensure energy production under varying environmental conditions, highlighting the adaptability and efficiency of cellular respiration across diverse life forms.
The Respiratory Chain: Coenzymes and Collaboration: Although Keilin and Warburg exchanged spirited debates on respiration throughout the 1920s and 1930s, Warburg inadvertently bolstered Keilin’s theory with his discovery of coenzymes—non-protein components vital to the chain. By the mid-20th century, the respiration process began to take form: glucose molecules break apart into fragments, entering the Krebs Cycle (a linked series of reactions), which sheds their carbon and oxygen atoms as waste (carbon dioxide, CO2) (see Figure 3). Hydrogen atoms from glucose bond with coenzymes and embark on their journey through the respiratory chain (see Figure 1).
The exact free energy changes at each step of the mitochondrial electron transport chain are as follows (see Figure 4) [5]:
- Complex I (NADH to ubiquinone): Approximately -16 kcal/mol of free energy is released.
- Complex II (FADH₂ to ubiquinone): Releases less energy compared to Complex I, approximately -4 kcal/mol.
- Complex III (ubiquinol to cytochrome c): Approximately -10 kcal/mol of free energy is released.
- Complex IV (cytochrome c to oxygen): The final step releases approximately -23 kcal/mol, as electrons combine with oxygen and protons to form water.
These incremental energy releases drive proton pumping across the mitochondrial membrane, creating the electrochemical gradient essential for ATP synthesis.
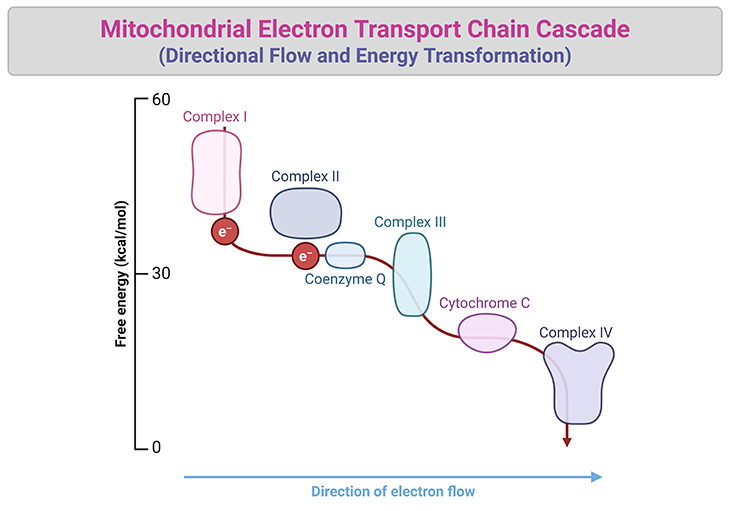
Figure 4. The Electron Transport Chain: Directional Flow and Energy Transformation. A comprehensive representation of the mitochondrial electron transport chain showcasing its cascade of energy-transforming reactions. Electrons (e⁻) from high-energy carriers, NADH and FADH₂, enter the chain at Complexes I and II, respectively. From there, they are transferred directionally to Complex III by ubiquinone (Coenzyme Q), a mobile carrier embedded in the inner mitochondrial membrane. Electrons then move to Complex IV via cytochrome c, ultimately combining with protons (H⁺) and oxygen to produce water. The directional electron flow is marked by incremental free energy releases at each step: -16 kcal/mol at Complex I, -4 kcal/mol at Complex II, -10 kcal/mol at Complex III, and -23 kcal/mol at Complex IV, totaling -53 kcal/mol. The released free energy drives proton pumping across the mitochondrial membrane, generating an electrochemical gradient essential for ATP synthesis in the ATPase (Complex V). All complexes are distinctly embedded within the membrane, while mobile carriers ubiquinone and cytochrome c shuttle electrons between them. This elegant molecular machinery illustrates the critical connection between electron transport and ATP production, a mechanism confounding scientists for generations before it was unveiled. {Cooper GM. The Cell: A Molecular Approach. 2nd edition. Sunderland (MA): Sinauer Associates; 2000. The Mechanism of Oxidative Phosphorylation. Available from: https://www.ncbi.nlm.nih.gov/books/NBK9885/}
Here, hydrogen atoms split into protons and electrons. Electrons traverse the chain via electron carriers, passing down like a current in an electrical wire. These carriers alternate between reduced (gaining electrons) and oxidized (losing electrons) states, creating a sequence of linked redox reactions. Each reaction releases energy—an exergonic process—ready to fuel cellular tasks. At the chain’s end, electrons meet oxygen and protons, forming water in a vital reaction mediated by Keilin’s re-named “cytochrome oxidase” (see Figure 1 and 4).
The Molecular Machines That Power Cells: Modern research reveals that the respiratory chain comprises four massive molecular complexes embedded in the inner membrane of mitochondria. Despite their enormous size relative to atoms, these complexes are barely visible under electron microscopes. Each complex is a mix of proteins, cytochromes, and coenzymes—a collaborative product of mitochondrial and nuclear genes. Astonishingly, a single mitochondrion hosts tens of thousands of these chains, which operate independently from one another, as do the complexes within individual chains.
The Unfinished Puzzle: Energy Conservation: While Keilin’s theory of the respiratory chain accurately described the stepwise flow of energy, one key question lingered: how is this energy conserved rather than dissipated? Scientists would soon uncover the answer in the form of ATP—the universal energy currency—a revelation that would redefine how we understand cellular power. Stay tuned for the next chapter of this story.
Take-Home Messages
- Respiration is a controlled biochemical process that extracts energy from glucose and powers all cellular functions.
- Respiration derives energy not only from glucose but also from other sources like fatty acids, amino acids, and ketone bodies, showcasing the versatility of cellular metabolism.
- The respiratory chain, composed of molecular complexes in mitochondria, enables stepwise energy release through electron transfer.
- Cytochromes and coenzymes are essential components of the chain, facilitating redox reactions and energy flow.
- Energy from respiration is conserved and transformed into ATP, the universal energy currency of life.
- This intricate process highlights the precision and efficiency of molecular machinery in sustaining life.
Summary and Conclusions
Respiration is the cornerstone of life, a masterfully orchestrated process that transforms potential energy stored in molecules into the vital force that powers every cellular function. At its heart, this intricate biochemical mechanism involves the breakdown of organic substrates—not just glucose, but also fatty acids, amino acids, and ketone bodies—demonstrating the metabolic versatility that sustains life under diverse conditions. These molecules are dismantled through a series of reactions, beginning with the Krebs Cycle, which extracts hydrogen atoms and releases carbon dioxide as waste. The hydrogen atoms, split into electrons and protons, enter the respiratory chain, a finely tuned molecular relay system embedded in the inner membrane of mitochondria.
Within this chain, electrons are passed between specialized carriers like cytochromes and coenzymes in a sequence of redox reactions. Each step releases energy in a controlled manner, much like the gradual flow of current through an electrical wire. This precision ensures that energy is not wasted in explosive bursts but is instead conserved for cellular use. The culmination of this process occurs when electrons and protons combine with oxygen to form water, completing a marvel of molecular efficiency. The respiratory chain’s massive molecular complexes, assembled from mitochondrial and nuclear genes, highlight the extraordinary interplay of cellular machinery and genetic collaboration.
But respiration does more than support basic survival—it drives every action, thought, and growth that defines life. The energy liberated is transformed into adenosine triphosphate (ATP), the universal energy currency that fuels everything from muscle contraction to neural signaling. By seamlessly converting potential energy into a form that is usable for work, respiration stands as a testament to the elegance and ingenuity of nature’s design.
As we unravel the complexities of this process, we gain not only a deeper appreciation for its precision but also insights that extend far beyond biology. The study of respiration informs fields like medicine, bioenergetics, and even sustainable energy solutions, offering profound implications for science and society. In its complexity, respiration captures the essence of life’s brilliance—a harmonious system, exquisitely tuned to power the vast symphony of existence.
For information on autism monitoring, screening and testing please read our blog.
References
- Ernster L, Schatz G. Mitochondria: a historical review. J Cell Biol. 1981 Dec;91(3 Pt 2):227s-255s. doi: 10.1083/jcb.91.3.227s. PMID: 7033239; PMCID: PMC2112799.
https://pubmed.ncbi.nlm.nih.gov/7033239/
https://discoveryandinnovation.com/papers/Schatz_1981.pdf - Slater EC. Keilin, cytochrome, and the respiratory chain. The Journal of Biological chemistry. 2003 Volume 278, Issue 19, 16455 – 16461
https://www.jbc.org/article/S0021-9258(19)58350-8/fulltext - Madeira VMC. Overview of Mitochondrial Bioenergetics. Methods Mol Biol. 2018;1782:1-6. doi: 10.1007/978-1-4939-7831-1_1. PMID: 29850991.
https://pubmed.ncbi.nlm.nih.gov/29850991/ - Gest H. Landmark discoveries in the trail from chemistry to cellular biochemistry, with particular reference to mileposts in research on bioenergetics. Biochemistry and Molecular Biology Education 2002;30:9-13
https://iubmb.onlinelibrary.wiley.com/doi/full/10.1002/bmb.2002.494030010004 - Protasoni M, Zeviani M. Mitochondrial Structure and Bioenergetics in Normal and Disease Conditions. Int J Mol Sci. 2021 Jan 8;22(2):586. doi: 10.3390/ijms22020586. PMID: 33435522; PMCID: PMC7827222.
https://pubmed.ncbi.nlm.nih.gov/33435522/
- Harrington JS, Ryter SW, Plataki M, Price DR, Choi AMK. Mitochondria in health, disease, and aging. Physiol Rev. 2023 Oct 1;103(4):2349-2422. doi: 10.1152/physrev.00058.2021. Epub 2023 Apr 6. PMID: 37021870; PMCID: PMC10393386.
https://pubmed.ncbi.nlm.nih.gov/37021870/